Fusion Breakeven Is a Science Breakthrough
But it is not a technology paradigm shift.
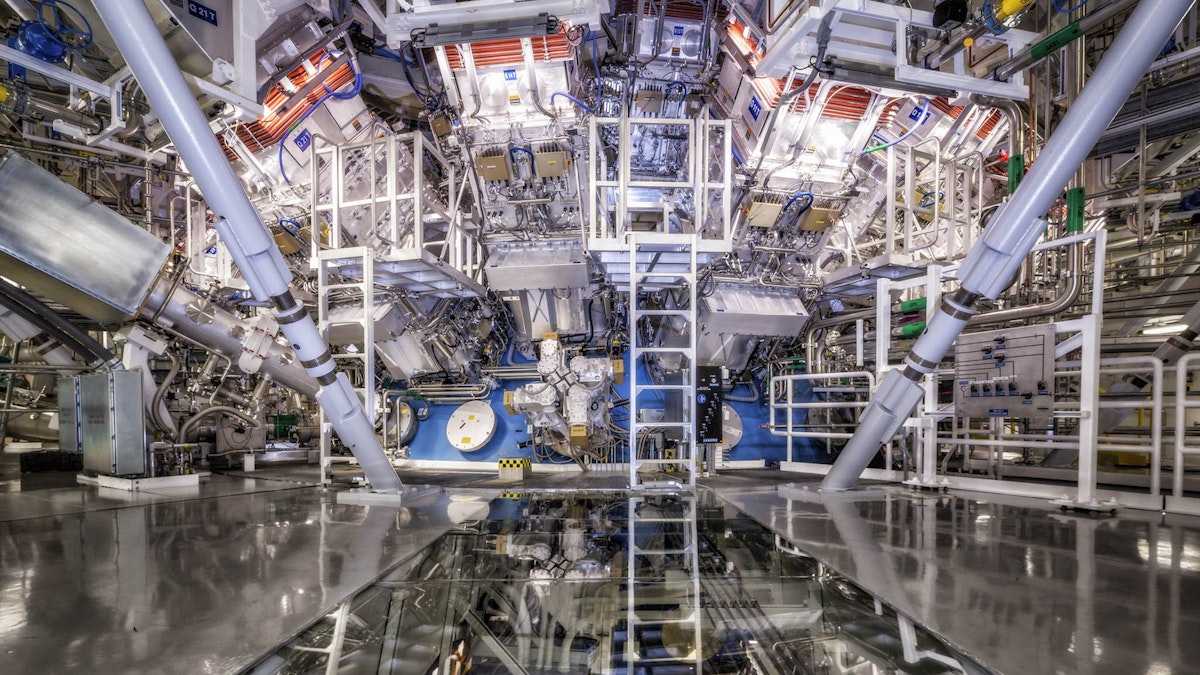
-
-
Share
-
Share via Twitter -
Share via Facebook -
Share via Email
-
Fusion has long been touted as the holy grail of energy production. Ever since it was first demonstrated in 1932 at the Cavendish Laboratory of Physics, University of Cambridge—where I am now working on a PhD—scientists and engineers have attempted to design and demonstrate fusion reactions that produce more energy than they consume, a concept known as a breakeven.
Last week, this long sought-after and ever-elusive breakeven goal was achieved. At a December 13th press conference, U.S. Secretary of Energy Jennifer Granholm, who was preempted by leaks to the press, confirmed that in the early hours of the morning on Monday, December 5th, researchers at the Lawrence Livermore National Laboratory's (LLNL) National Ignition Facility (NIF) achieved net energy gain through a fusion reaction. This is a major scientific milestone.
While the achievement should be celebrated, commercial fusion (much less the unlocking of a limitless energy source, as overzealous articles have described the experiment) is far from reality. Understanding why, though, will help show how we could really get to that bright future.
What is nuclear fusion?
Fusion is a nuclear reaction where two lighter nuclei—the particles that make up the dense center of an atom—fuse together, forming a heavier nucleus of a different element. The mass of that heavy nucleus is ever so slightly less than the sum of the mass of the two original nuclei. The missing mass is converted to energy. And a lot of it.
Sounds great, but the process of getting the nuclei to fuse is difficult. Nuclei are positively charged and repel each other. But if two nuclei come very close together, the strong nuclear force overcomes the repulsion, and the nuclei fuse. In order to bring two nuclei close enough to fuse, a lot of energy needs to be added to the system. The central aim of fusion experiments, like the one at NIF, is for the output fusion energy to exceed the input energy.
There are many approaches to fusion. The two broadest categories are magnetic confinement fusion (MCF) and inertial confinement fusion (ICF).
MCF aims to sustain fusion for long periods of time at low densities and pressures, whereas ICF reactions occur for a very short period of time but at extremely high pressures and densities. The former confines extremely hot plasmas of fuel using strong magnetic fields to prevent hot plasmas from cooling off and expanding. MCF achieves fusion at sufficiently high temperatures, ideally in a sustained reaction. The latter concept is ICF, which NIF employs. That process compresses and heats fuel—located inside of a special container, called a target assembly—achieving fusion at high densities and in short bursts. The most common fuels for fusion are isotopes of hydrogen, deuterium, and tritium due to those having the highest probability of fusing.
For decades, scientists have performed various permutations of MCF and ICF at facilities around the world, such as the Joint European Torus (JET) in the United Kingdom, Tokamak Fusion Test Reactor at the Princeton Plasma Physics Laboratory, the NIF itself, and others. But until now, none of these experiments have achieved fusion reactions that have produced more energy than was put into the system—that is, scientific breakeven (or when scientific gain equals one).
The concept of gain is the subject of much confusion, especially when comparing ICF and MCF technologies. Gain is a ratio that must be contextualized with respect to the boundaries of the system under review. In general, the scientific standard for gain is the energy produced by a fusion reaction divided by the externally supplied energy at the boundary of the plasma for the given fusion approach.
In the case of ICF, this boundary would be drawn at the target, hence, scientific gain would be synonymous with gain in the target, in which laser energy is transferred to the fuel capsule. For MCF, this boundary is at the confinement edge of the plasma, which is defined by the magnetic fields.
Fuel gain, as opposed to scientific gain, uses the energy actually absorbed by the fusion reactants (e.g. deuterium and tritium fuel) as not all delivered energy is absorbed by the fuel.
Another concept in reporting on fusion that tends to get confused is “ignition,” which technically refers to when the plasma retains enough heat from the fusion reaction to sustain itself, so external energy required to sustain the reaction goes to zero, and gain goes to infinity. For example, in MCF, reaching ignition would indicate that a fusion reaction would endure until there is not enough fuel or containment is lost. But multiple articles and reports have misunderstood the difference between scientific gain and ignition because of differences between MCF and ICF.
This particular point of confusion has arisen because, in August 2021 and in the just-announced results, NIF did produce self-propagating fusion, whereby fuel in the hot-spot triggered fusion reactions in surrounding fuel. But that does not mean that external energy supplied to the target was zero. It was far from zero. In the August 2021 experiment, it was 1.9 MJ, and in the December 2022 experiment, it was 2.05 MJ.
In this way, these two NIF results could be said to have achieved ignition in the fuel for an exceedingly short period of time. But since the NIF is a pulsed fusion experiment, where fusion reactions last on the order of 100 trillionths of a second before the plasma expands out of fusion conditions, it inherently requires external energy from laser heating to ignite and cannot self-sustain. The term ignition, while not scientifically incorrect with reference to the fuel, likely adds to the confusion. Breakeven or target gain are more helpful descriptors.
What Exactly Happened at NIF?
As Secretary Granholm announced, a recent fusion shot experiment at NIF achieved energy gain, surpassing breakeven. The reaction yielded 3.15 megajoules (MJ) of energy from 2.05 MJ of laser energy incident on the target, resulting in a scientific or target gain of 1.54 (or 154 percent) more energy output than delivered to the target.
But how do we understand what that means? Not only are there different ways of defining gain, as discussed above, but the NIF reactor itself must also be better understood.
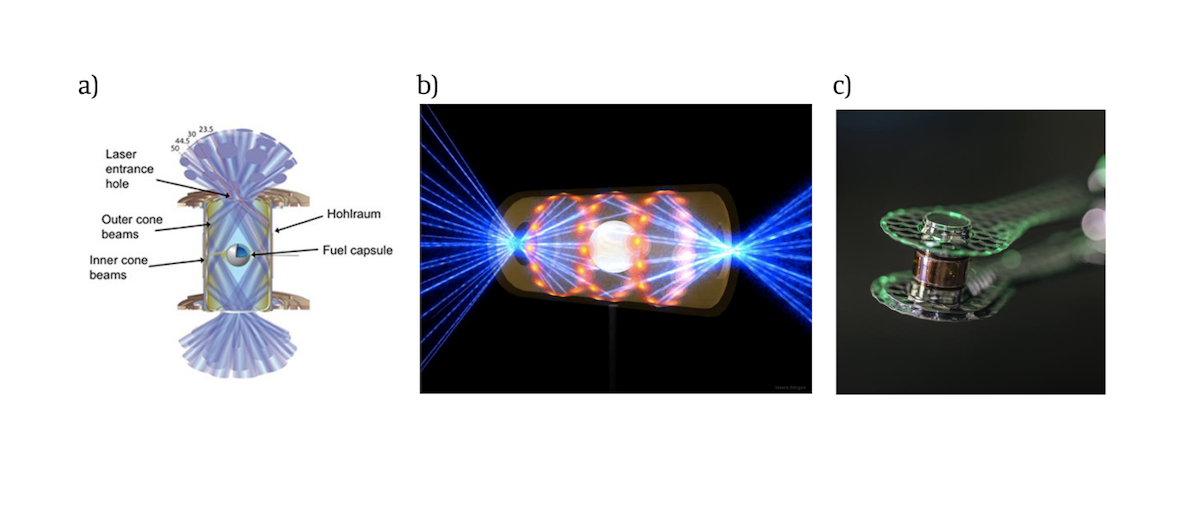
The NIF is an indirect-drive laser-induced ICF system with the world’s largest laser array. In NIF fusion experiments, 192 ultraviolet (UV) lasers, pulsed for a few billionths of a second at a time, are focused within a metal target assembly through openings at the end of a hohlraum (usually a gold or uranium tube containing a hollow, spherical fuel capsule about the size of a peppercorn).
The hohlraum subsequently converts the laser energy to x-ray energy, radiating a uniform bath of x-rays within the tube and penetrating the surface of the fuel-containing diamond capsule called the ablator. The x-rays produce an ionized plasma on the outer layer of the ablator, which explodes, sending shock waves toward the center of the capsule.
Meanwhile, the rest of the capsule, and the inner lining of frozen deuterium and tritium, implode under ablation pressures on the order of hundreds of megabars and at significant velocities of roughly 400 kilometers per second, forming a “hot-spot” where conditions are suitable for fusion. For comparison, this implosion is the equivalent of compressing a basketball to the size of a pea, all while ensuring the sphere is symmetric throughout compression.
During the rapid implosion, roughly 10 percent of the fuel undergoes a process called stagnation, where the immense kinetic energy is converted to extreme temperatures of roughly 150 million degrees celsius and pressures more than twice those of the center of the sun, and triggers a propagating fusion reaction. An important note on terminology is that a burning plasma occurs when self-heating caused by the fusion reaction—in this case from the implosion—exceeds external heating. Whereas, an ignited plasma is when self-heating caused by the fusion reaction exceeds all losses of heat from the plasma, which is necessary to achieve a self-perpetuating fusion reaction. After the fuel burns or ignites, the fusion plasma expands, and energetic neutron emissions from the fusion reaction explode away. Those could, in principle, be harnessed for energy conversion in a practical power plant design.
Just last year, NIF made headlines for achieving a plasma burn that yielded 1.3 MJ from 1.9 MJ of input energy, which was at the time a world-record target gain of 0.7 (or 70 percent), falling just short of scientific breakeven. What was particularly important about the achievement a year ago was that the fuel ignited, laying the groundwork for this month’s breakeven experiment. But the August 2021 results were not easily reproducible and highly dependent on capsule quality.
The notable achievement from the December NIF test was the first demonstration of scientific breakeven, at a target gain of 1.54 (154 percent more energy out than put in), excluding demonstrations of thermonuclear fusion nuclear weapons, which are not controlled laboratory reactions.
This recent fusion breakthrough campaign began with a September 2022 experiment, which yielded 1.22 MJ of fusion energy and featured two key improvements from the August 2021 fusion attempt, despite having worse capsule quality, in which even minute (smaller than the diameter of a human hair) defects can result in failure. First, the NIF substantially increased laser energy from 1.9 MJ to 2.05 MJ. Second, the experiment used a thicker ablator capsule layer, which improved the margin for reaching ignition and doubled the proportion of deuterium and tritium fuel burn (i.e. consumption), from 1.9 to four percent. For the recent successful experiment that realized scientific gain, energy among the 192 lasers was redistributed to improve the symmetry of implosion.
When could “limitless energy” through fusion become reality?
Practically, commercial fusion is still far off.
Despite NIF’s accomplishment, the scientific gain discussed here only takes into account the energy delivered to the target by the laser instead of the electrical energy needed to power the laser array itself, which is an inefficient process. Engineering gain, which defines the total electrical energy output from fusion divided by the total electrical energy input to heat the fusion fuel, is the relevant but non-scientific metric for practical commercial fusion.
NIF’s UV lasers use inefficient infrared amplification because they were low-cost options at the time of design and construction in the 1990s. In the announced NIF experiment, roughly 300 MJ of electrical energy was consumed to generate only 2.05 MJ of laser emission energy to trigger fusion. That 2.05 MJ of laser energy produced 3.15 MJ of fusion thermal energy output. The result is only a ~0.0105 (or 1.05 percent) engineering gain, not including the conversion of fusion thermal energy to electrical energy. The laser system itself only operates at a ~0.68 percent efficiency. State-of-the-art solid-state lasers could operate at 10 to 20 times that efficiency, but that is nowhere near efficient enough to reach breakeven engineering gain.
Conversion of thermal fusion energy to electrical energy for consumption would undergo further losses in the range of perhaps 20 to 40 percent. For a NIF-like design to be practical for energy applications, and ignoring economic considerations, fusion target gains would likely need to reach the range of 20 to 80 (or 2,000 to 8,000 percent) instead of the announced 1.54 (or 154 percent).
Further, NIF can run experiments like the one it announced infrequently (no more than a few per day in the extreme), but for a NIF-like fusion design to be a practical power plant, such fusion reaction events would be required to run at roughly the equivalent of ten times per second.
NIF’s primary function is not even related to energy production at all. Rather, NIF is an experimental facility funded by the National Nuclear Security Agency’s Stockpile Stewardship Program to study fusion reactions for nuclear weapon applications and to ensure effective nuclear deterrence. NIF is essentially an alternative to underground nuclear tests, the last of which in the United States occurred in 1992 and which officially ended in 1996 after the signing of the comprehensive Nuclear-Test-Ban Treaty in 1996. In the first few years after NIF first came online in 2009, it was expected that reaching ignition would be straightforward, explaining the National Ignition Facility’s namesake.
But it wasn’t. Getting to ignition has been anything but straightforward. Celebration of the LLNL fusion scientists’ efforts is certainly in order, but fusion has a long path ahead to practical commercial energy generation. The history of thermonuclear fusion is a cycle of excitement about new designs and promising experiments, followed by a humble return to the reality of the immense difficulty of controlling super-hot plasmas. We should expect this process to continue as the field makes steady progress in its efforts to unlock fusion energy for society.