Emissions In Reverse
Net Zero Isn’t Possible Without Carbon Capture, Utilization, and Storage. Here’s How to Get the “Use” Part Right.
-
-
Share
-
Share via Twitter -
Share via Facebook -
Share via Email
-
Last November, the 2021 United Nations Climate Change Conference (COP26) culminated in the Glasgow Climate Pact. Signed by nearly 200 nations, the pact, which reaffirms the goal of limiting global warming and finds that the commitments currently in place to cut greenhouse gas emissions will not be enough to hit global targets, will dictate much of the worldwide agenda on climate change for the next decade.
In it, the focus will be on achieving net-zero emissions by 2050. There are two main routes for doing so: either emitting no further greenhouse gases or removing what has already been released by human activity into the atmosphere. The second may sound far-fetched, but it is the premise of afforestation and reforestation, efforts with long histories. And on the higher-tech end, it is also the principle behind solutions like carbon capture, utilization, and storage (CCUS).
A suite of technologies that captures and removes carbon from the environment, CCUS has real potential to get the world to negative emissions. It can be applied to ongoing sources of carbon dioxide, like power generation and industrial processes, to prevent the release of new carbon into the atmosphere. There are also technologies that remove carbon emitted into the air long ago and, in doing so, reduce overall accumulation.
Since CCUS can help minimize emissions from energy systems that already exist, it balances the need to protect the environment with the importance of high-emitting and energy-intensive sectors to economies around the world.
We can’t, in fact, get to net-zero without it, as evidenced by research from Columbia University’s Center on Global Energy Policy and the Intergovernmental Panel on Climate Change. A graph from the Global CCS Institute, a think tank promoting carbon capture and storage, confirms this point.
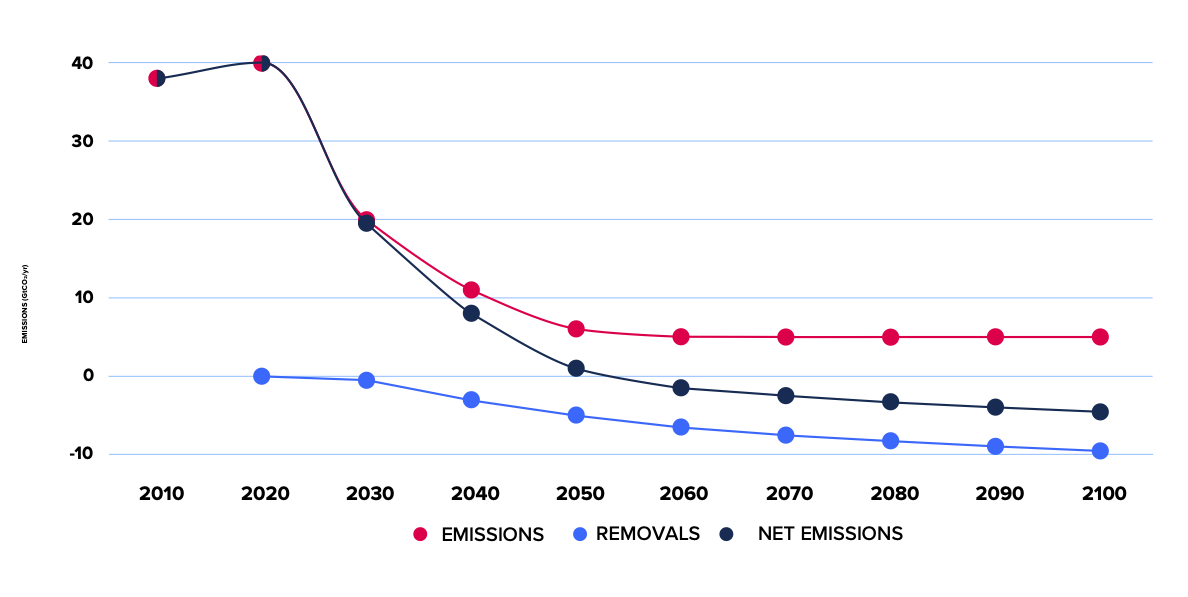
It should not be surprising, then, that the Glasgow Climate Pact calls on signatories to accelerate the development and deployment of technologies like CCUS. And inasmuch as the pact’s goal is to achieve net zero by 2050—and in a way that is not harmful to human prosperity or the needs of developing countries—it was right to do so.
How It Works
There are three main methods of carbon capture: pre-combustion, post-combustion, and oxy-fuel combustion. Briefly, pre-combustion capture refers to removing the carbon from fossil fuels before the fuel is burned. In this process, the fuel is converted into a synthesis gas consisting of hydrogen and carbon monoxide. The hydrogen is separated and burned, and the carbon monoxide is converted into carbon dioxide, which can be compressed and liquefied for storage.
Post-combustion capture occurs after the fuel has been burned. In this process, carbon dioxide is captured from exhaust gases and removed. That can happen through chemical absorption, membrane separation, or pressure swing adsorption.
Lastly, oxy-fuel combustion capture involves burning fuels in pure oxygen, so that the exhaust is mainly carbon dioxide and water. The carbon dioxide can then be purified, compressed, and transported.
Compared to other methods, oxy-fuel provides the easiest removal of pollutants. But it also requires stringent safety management and involves high maintenance costs. Further, it consumes more fuel than the other options, decreasing its net efficiency.
Pre-combustion capture is the most energy-efficient, but its processes are more complex and difficult to apply than post-combustion capture, and the capital investment tends to be larger.
Post-combustion capture, meanwhile, offers high levels of operational flexibility, lower technology risks, and the integration of renewable technologies into the process. Though it is less energy-efficient than pre-combustion capture, its capital costs are lower. As a result, it is the most widely used of the CCUS technologies.
Whether pre-, post-, or oxy-fuel combustion, most CCUS technologies can absorb around 85 percent to 95 percent of carbon dioxide produced by a power plant. And even given the added energy needed to run CCUS systems, which lowers their efficiency, individual CCUS technologies have been reported to reduce carbon dioxide emitted by more than 85 percent where they are deployed, with some projects at 90 percent and even 100 percent.
Once captured, if not used onsite, the emissions are generally transported by pipeline or ship (or, if amounts are small, by rail or even truck) for use elsewhere or to permanent storage sites. Pipeline transport tends to be most efficient, economical, and common. The risk of leak or failure is low, on par with that from oil and gas pipelines, which are safely managed. But the viability of pipeline transport depends on the conditions and location of the operation, as well as the size and configuration of existing pipelines. Feasibility depends on transport distance and volume of gas being transported too. In early stages, projects can be expected to face relatively high transport costs until throughput increases as the industry grows.
Helping matters is that there is already extensive pipeline infrastructure around the world to support CCUS, 85 percent (or 5,000 miles) of which is in the United States alone. In addition to pipelines specifically for carbon built since the first large-scale capture and storage facility was commissioned in the early 1970s, there is a possibility that existing oil and gas pipelines could be retrofitted for CCUS use. The Global CCS Institute expects that the demand for added transportation capacity will “unfold in an incremental and geographically dispersed manner” as the deployment of CCUS expands, which means that it should be easier to meet.
Use It Or Lose It
Of course, once carbon dioxide is taken away from where it is created, something has to happen to it. And that means utilization, storage, or something in between.
First, utilization. Carbon can be used directly, as in carbonated beverages, or as feedstock for industrial chemical processes—for example, in the creation of concrete or production of synthetic fuels. A New Jersey-based start-up, for example, is producing a cement that has the capacity to do away with at least 1.5 gigatonnes of carbon dioxide annually while also saving fresh water and reducing energy consumption. (For context, estimated global concrete emissions in 2019 were just over 1.5 times that amount, at 2.3 gigatonnes.)
And large-scale industrial products aren’t the only thing in play. In November 2021, the Canadian think tank SecondStreet.org released a policy brief documenting some of the innovative ways businesses around the world are using captured carbon for consumer-facing services and products, like vodka and hand sanitizer (produced by a New York-based company) and soaps and crayons (made in Calgary). While not necessarily scalable at this stage, these sorts of examples are proof that captured carbon can be used in marketable consumer goods.
Second, there are applications for carbon dioxide in between use and storage. For several decades, for example, energy firms have injected carbon dioxide into operating oil fields in enhanced oil recovery (EOR) projects. The process boosts the amount of oil that can be extracted by an additional 15 to 20 percent of the original oil in the rock formation and traps 90 to 95 percent of the injected carbon dioxide underground.
In 2016, EOR was responsible for about 5 percent of total US crude oil production. There are doubts about whether carbon dioxide applied this way—to the production of more carbon-emitting fuel—reduces overall emissions. However, since most of the injected carbon dioxide does remain trapped, it at least lowers the carbon intensity of the oil produced, for a projected 63 percent net reduction in emissions for each barrel produced. Studies have also shown that each barrel of oil produced in this fashion displaces other production sources with higher carbon dioxide intensities, further depressing emissions.
With estimates showing a growing global demand for oil through 2040, driven largely by developing economies, the world should not ignore the proven potential of EOR. In the United States, the Congressional Research Service projects that the majority of CCUS projects in the near future will continue to be EOR, to offset the costs of carbon capture and generate revenue. Already, the United States uses about 32 million tons of carbon dioxide per year for this purpose and accounts for nearly 90 percent of the world’s EOR oil production.
Unfortunately, in the context of climate change mitigation, there is a disconnect between regions with significant potential for EOR and regions with the greatest anticipated need for it, owing to their population growth and continued dependence on fossil fuels. The Middle East, North Africa, and the United States all have high EOR potential, primarily due to the presence of depleted oil reservoirs that have the necessary characteristics to store carbon dioxide. Yet it is countries like China and India that would likely have the greatest need, given their booming demand for fossil fuels.
In addition, low oil prices and relatively high carbon dioxide prices make EOR less economically viable. In the United States, at oil prices of approximately $100 per barrel, carbon dioxide needs to be available for $45 or less per tonne. So, efforts to raise the price of carbon dioxide will work against large-scale EOR.
What all this means is that, although there are more and more options for using carbon directly or using and storing it through EOR, emissions will more commonly continue to be holed away permanently.
Carbon In Store
In addition to the kind of storage that occurs through EOR, captured carbon can be sequestered in salt water-filled geologic formations deep below the surface, both onshore and off. According to Howard Herzog, a senior research engineer in the MIT Energy Initiative and author of the book Carbon Capture, appropriate sites meet four main criteria: high porosity with good permeability (that is, rock with ample air spaces through which liquid can easily move); deeper than 800 meters; an impermeable caprock that seals the site; and a caprock that is thick and continuous over large areas. A multitude of these storage sites have been identified worldwide. According to an Intergovernmental Panel on Climate Change special report, when appropriately selected and managed, the amount of carbon dioxide retained is “very likely to exceed 99% over 100 years and is likely to exceed 99% over 1000 years.”
Geologically, the storage of carbon dioxide is the mirror image of the extraction of oil and gas, and is possible thanks to half a century of oil industry expertise. There are four main mechanisms that work together to keep the carbon sequestered. In structural trapping, the injected liquid carbon dioxide migrates upward through porous rocks until it is stopped from going farther by the caprock, preventing its escape. In residual trapping, carbon dioxide moves through porous rock, which acts like a sponge, displacing existing fluid. Solubility trapping occurs when the injected carbon dioxide dissolves in salt water (brine) already present in the rock, making a weak carbonic acid that is denser than the surrounding fluids and sinks to the bottom of the formation over time, trapping it securely. Finally, mineral trapping results from the reaction of carbonic acid with the surrounding rock, which binds carbon dioxide to the rock and forms solid carbonate minerals. This permanently traps and stores that portion of the injected material.
In EOR, for example, although a small amount of carbon dioxide comes back to the surface with the oil, more than 90 to 95 percent of the carbon dioxide injected is permanently sequestered. Even a decade ago, scientists projected that approximately 136 million metric tons could be stored in US EOR fields alone. If actually captured and stored by 2025, that would account for over 10 percent of the amount required to be removed from the atmosphere to meet Paris Agreement climate goals.
And this storage amount—136 million metric tons—is an underestimate. First, it refers only to the carbon that could be stored by EOR. Second, deep saline formations are widespread and have larger storage capacities than oil and gas reservoirs. Estimates suggest that known deep saline formations in the United States have the potential to store more than 12,000 billion tonnes of carbon dioxide, which well surpasses the amount required to meet current climate goals. However, these formations tend to be less understood than oil and gas fields and therefore have greater associated risks.
For example, the very presence of an oil field indicates the existence of an effective caprock (or else the oil would have already escaped). But a deep saline formation will require testing and modeling to determine the same. In addition, for a mature oil field, the depth and porosity of the formation are often known, and there is generally already infrastructure that can be put to use to inject carbon back into a site. For saline formations, none of that yet exists, which adds to the cost. So, while deep saline formations have larger capacities, it is often the same formations from which oil and gas are extracted that are most economical for storage.
Getting to Net Zero
It is hard to deny the benefits of CCUS. Yet implementation lags potential.
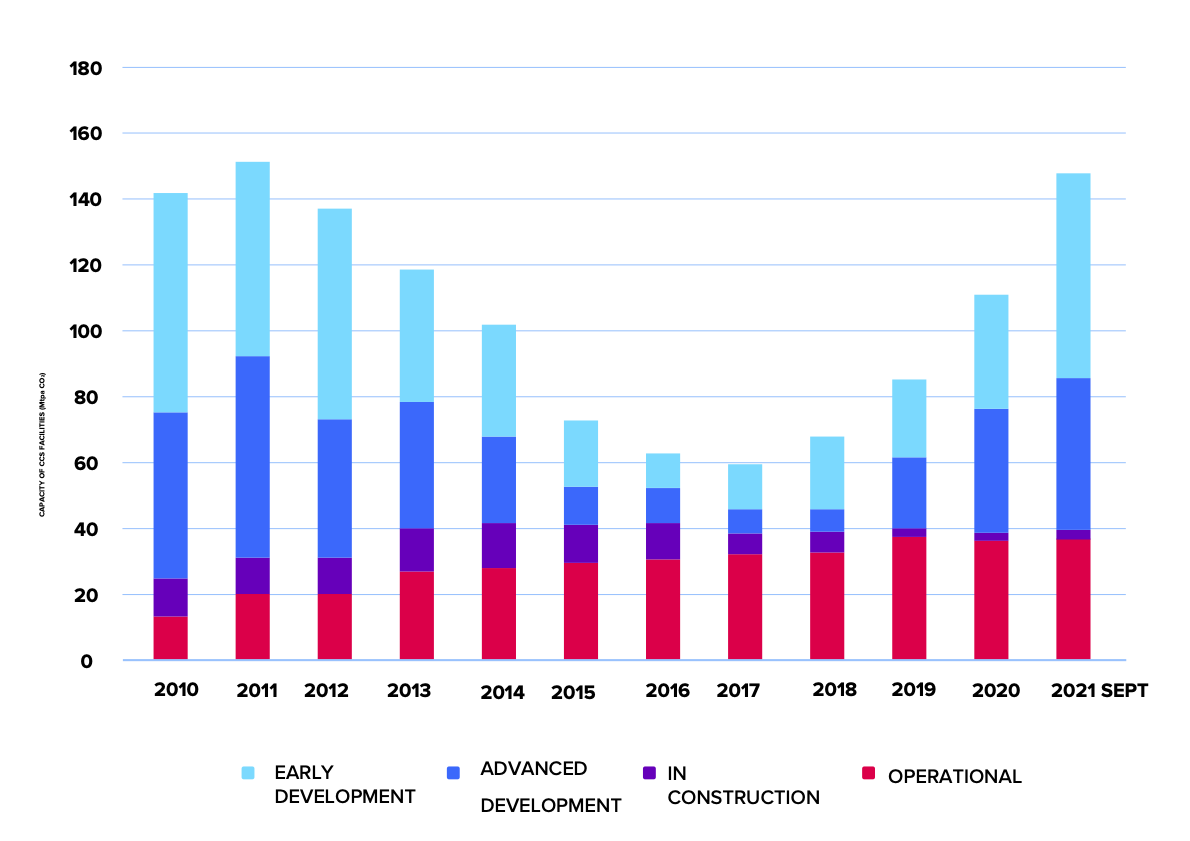
To be sure, there has been plenty of progress. Since 2017, there has been a growth in facilities in early or advanced development for carbon capture and storage, many of which are in the United States. With a long lead time, it takes a while for these facilities to become operational and then reflected in the data; but from January 2020 to August 2021, close to 50 new US projects were announced, which will double the capacity of commercial carbon capture and storage projects in operation or development.
Yet even those won’t be enough. Net-zero targets call for the annual capture and storage of 1150 million tons of carbon dioxide per year by 2030, and the facilities currently in construction, development, and operation are expected to account for around 13 percent of this. Of these, 22 percent are EOR.
Ramping up won’t be easy. A host of intersecting policy and regulatory frameworks—local, national, international—are at play. Every case will be different, but a broad assessment of incentives and regulations like one 2019 study in the journal Resources is useful. As the study’s authors write, it is not just economics that determines CCUS deployment. There are organizational, technological, ecological, social, and political-legal factors as well.
At the broadest level, geography matters. Some places simply have more storage than others. Then there’s economic development since CCUS projects are expensive and complex. There is both more demand and more expertise on hand where traditional energy players are strongest. Trade relations play a role too. CCUS technologies can be stood up faster when there is an open exchange of knowledge and technological components.
Beyond that is politics. In some cases, there’s more political appetite for solutions when emissions are highest. So far, most deployment has occurred in high-emitting countries. General public interest in such projects helps, as does fear about high unemployment rates related to the transition from fossil fuels, since CCUS technology can create jobs in fields where workers are losing theirs. Public interest concentrated around other environmental or green solutions can hinder CCUS, as can the public perception that CCUS is a Trojan horse for continued fossil fuel use.
All of this informs any given project’s regulatory environment. Where reduction of greenhouse gas emissions is the main goal, CCUS is appealing. Where monitoring or reporting (as in a permitting system) or coercive and remediation measures (such as carbon taxes) are the focus of environmental policy, deployment of such systems is far harder. For example, lack of a carbon dioxide storage-permitting process has been a critical regulatory challenge around the world.
Within the patchwork of factors that determine CCUS deployment, it turns out that the United States fares relatively well, and according to the Global CCS Institute, 36 of the 71 projects added in the first nine months of 2021 were in the United States. That’s because the United States has a set of developed, stable, and clear regulations that promote deployment along with well-established funding, incentives, and private-sector involvement. Canada is also a leader in this area, and in recent years has deployed some of the world’s largest and most advanced carbon capture projects. As countries around the world seek ways to meet emissions targets, the United States’ and Canada’s already well-developed sectors will give them an edge.
We know that we must do something to limit greenhouse gas emissions—this has been established time and time again. Carbon capture, utilization, and storage technologies present an opportunity to do so in order to strategically mitigate climate change and help the world get to net-zero commitments without derailing future growth. But to get there, policy makers around the world must remember that the net-zero goal applies to global emissions, which will mean finding CCUS options appropriate to myriad conditions. Countries with the capacity and potential for such technologies should, to the extent possible, do as the United States has done and create the business case for investment, so that adoption starts yesterday.