Advancing Nuclear Energy
Evaluating Deployment, Investment, and Impact in America's Clean Energy Future
-
-
Share
-
Share via Twitter -
Share via Facebook -
Share via Email
-
Executive Summary
Leadership in new nuclear technologies will powerfully benefit America’s energy future. Advanced nuclear reactors are versatile, reliable, long-lasting, land-efficient, resource-efficient, geopolitically secure, and scalable sources of clean energy. Bold investments in advanced nuclear technologies in the United States will advance technological innovation, secure US leadership in international nuclear markets, and support national energy security and electricity grid resilience, all while improving environmental health and accelerating US climate action.
However, forging a promising future for the domestic advanced nuclear sector will require increasing investment and policy support. Such efforts will generate far-reaching national benefits in both the near-term and long-term.
This report uses a high-resolution nationwide model of the United States electricity sector to demonstrate how advanced nuclear reactors might play a major role in a least-cost plan to transition the power grid entirely to clean energy sources by 2050, assuming that the first advanced reactors are available for deployment by 2030. A range of input assumptions were developed to encompass uncertainty in cost and learning rates to estimate the outer bounds of potential future deployment. Across these scenarios, the model chooses to deploy a large quantity of advanced nuclear power plants (Figure ES-1). Even in the case that first-of-a-kind advanced reactors are deployed at the high end of current cost estimates and benefit from very little technological learning as additional units are deployed, advanced nuclear captures a significant share of future electricity generation. This finding indicates that advanced nuclear energy technology provides important and extremely valuable benefits to the electricity system.
In particular, advanced nuclear reactors efficiently complement other clean energy technologies like wind and solar power, balancing out variations in generation over time to reliably meet US electricity demand. The flexibility of advanced nuclear power can produce long-term cost savings as America transitions to a clean energy system.
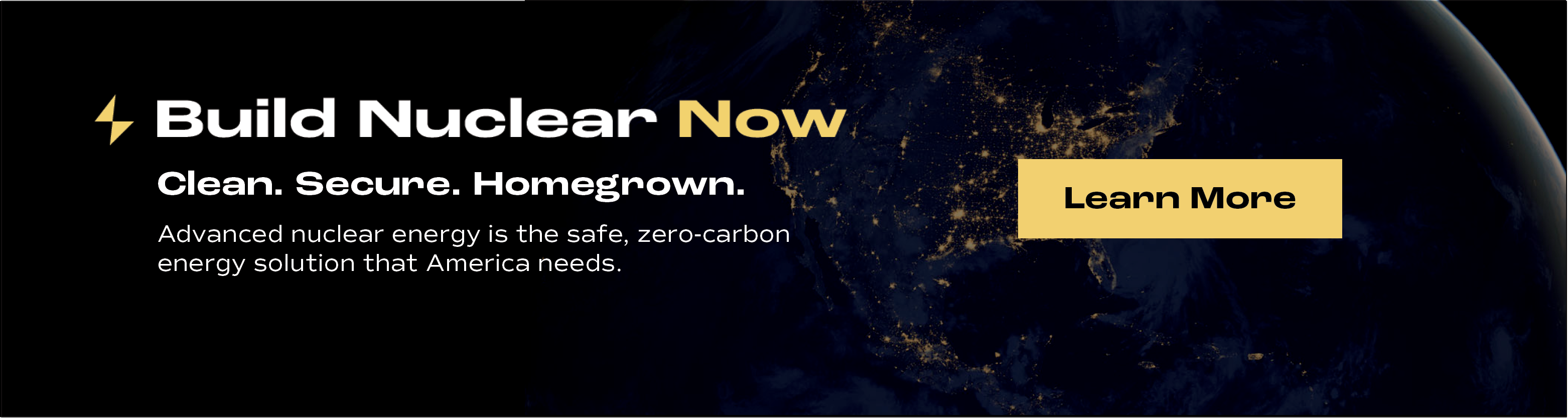
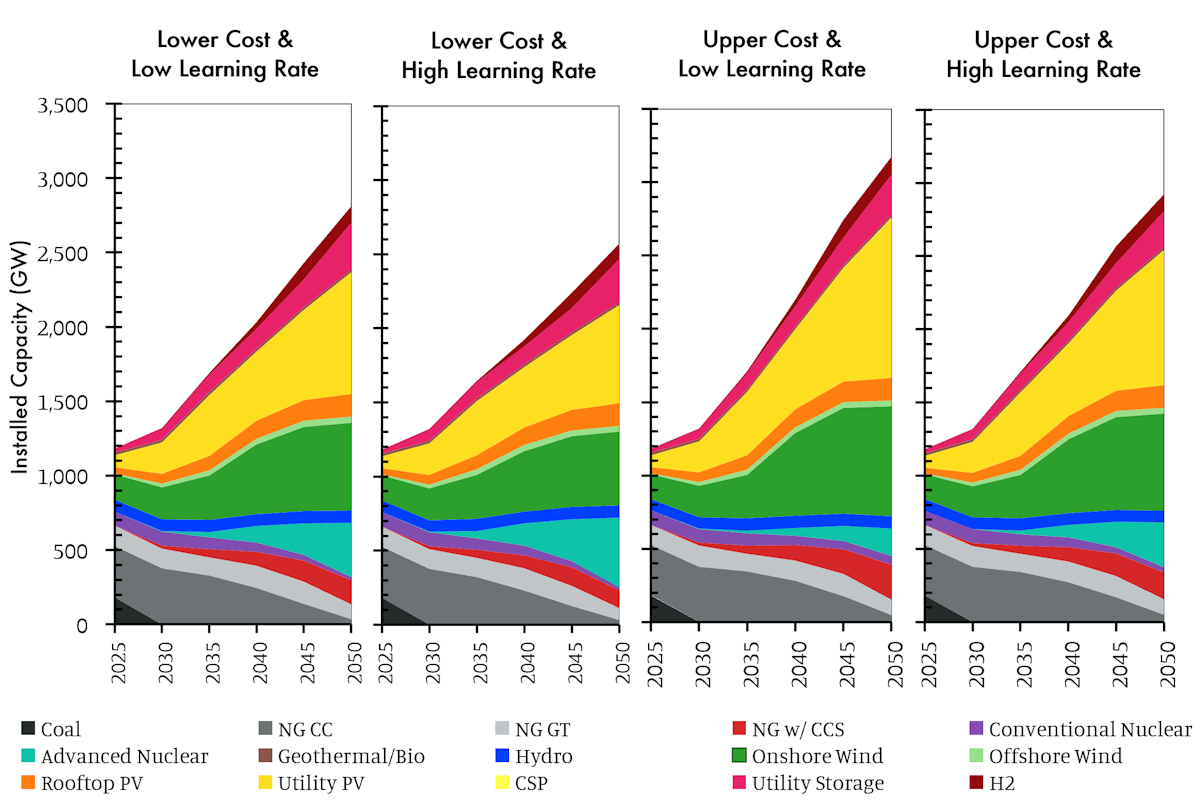
Advanced Nuclear Deployment and Capital Investment:
This modeling study shows that a US clean energy transition incorporating advanced nuclear energy could require cumulative capital investment for advanced nuclear power plant construction on the order of $150 to $220 billion by 2035, growing to a total of $830 billion to $1.1 trillion by 2050 (Figure ES-2). Early capital investment and learning-by-doing lead to substantial reductions in project costs and levelized electricity costs for advanced nuclear technologies, resulting in the large-scale nationwide deployment of new reactors.
Widespread commercial deployment of advanced reactors in this study starts in the early-2030s and rapidly accelerates as the electricity sector grows over time, potentially supplying around 20-48 percent of domestic clean electricity generation in 2050, which would be 1,400 to 3,600 terawatt-hours per year (TWh/yr). Total domestic deployed advanced nuclear capacity reaches 19 to 48 gigawatts-electric (GWe) in 2035, reaches 54 to 150 GWe in 2040, and grows to 190 to 470 GWe by 2050.
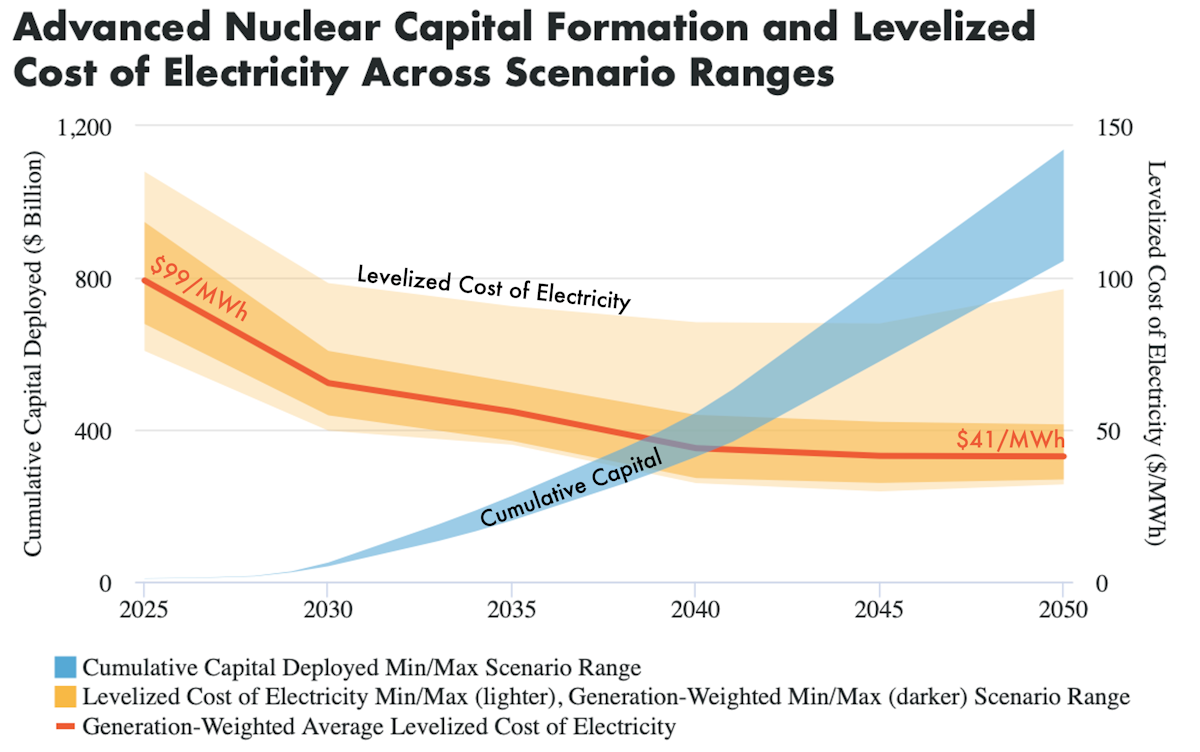
Economic and climate benefits of advanced nuclear energy deployment potentially include the following:
- A United States clean energy transition pathway that incorporates advanced nuclear power can help reduce the costs of a future national clean energy system.
- Low-emissions heat and steam from advanced nuclear plants can supply reliable, clean energy for hard-to-decarbonize sectors such as heavy industry and chemicals.
- Clean advanced nuclear reactors can repower fossil-fuel power plants using readily available infrastructure, increasing economic investment, and promoting a just transition for local communities.
- A successful future nuclear sector will produce new job opportunities in the manufacturing, construction, operation, and maintenance of nuclear plants, creating between 74,000 and 223,000 permanent jobs in operations and maintenance by 2050 alone.
- Successful demonstration and commercialization of advanced nuclear power will competitively position the United States as a clean technology leader at a critical moment in the global clean energy transition.
Barriers to advanced nuclear energy deployment can potentially be overcome through the following:
- Immediate capital investment can enhance the potential for cost reductions and the total domestic market opportunity.
- Avoiding cost overruns on early projects and making cost improvements over time will increase the speed and magnitude of advanced nuclear deployment.
- Developing supply chains for fuel and component manufacturing is essential for the broad deployment of advanced nuclear reactors.
- New federal regulatory frameworks currently being formulated, and streamlining of existing rules, will be key to ensuring timely licensing and construction of new advanced nuclear projects.
- Lifting state-level moratoria and restrictions on nuclear projects will expand market opportunities and attract new capital investment to states that reform existing legislation.
Opportunities for public policy support include the following:
- Federal loan guarantees.
- Environmental impact pre-qualification and feasibility studies.
- Regulatory licensing modernization and fee reform.
- Technology-neutral clean energy tax credits.
- Inclusion of nuclear energy in state clean energy portfolio standards.
- Support for export of advanced nuclear projects.
Future Outlook:
Advanced nuclear reactors can play a key role in cost-effective decarbonization of the national power sector, reliably supporting a high-renewables energy system. Updated, realistic cost assumptions and accurate operational characteristics reveal that advanced nuclear technologies provide high value for a clean electricity grid and possess significant market potential. Emerging advanced nuclear technologies will ultimately compete in the marketplace based on cost, operating parameters, and the ability to meet diverse customer needs, shifting the balance among which technologies become dominant. Early advanced nuclear deployments may be most competitive or efficient for specific target markets and customers, including existing nuclear power sites, sites with retiring fossil fuel plants, remote or island communities, and military installations. As advanced reactor deployment expands, however, cost improvements over time, and the need for firm energy that provides the required operational characteristics can drive large-scale nationwide adoption in support of a wider affordable clean energy strategy.
Download the Full Report >>>For access to the appendix and references, please download the PDF version of the report above.
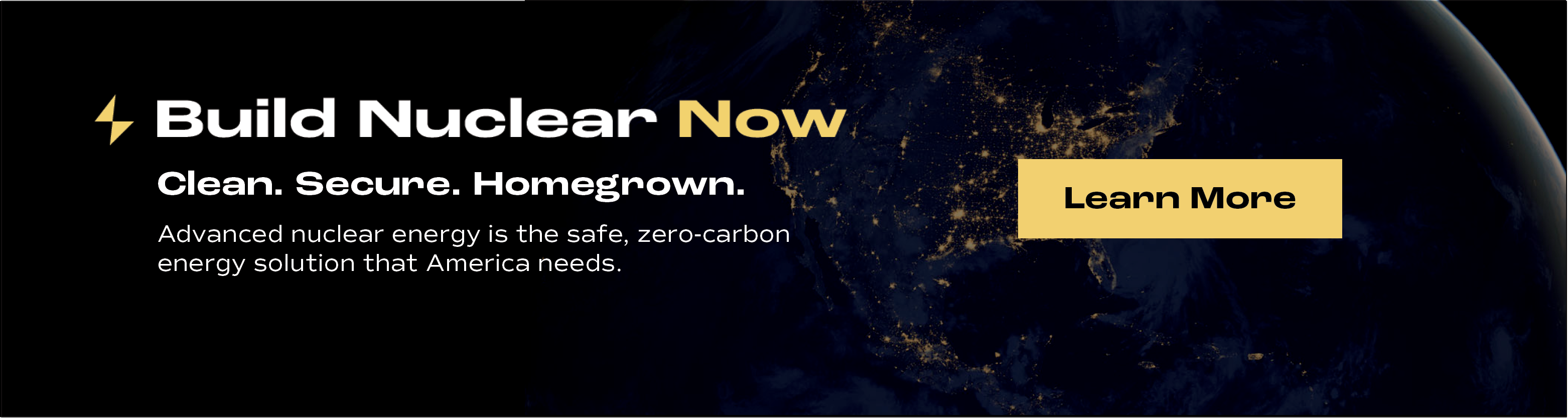
Introduction
We currently live in an era of rapid technological innovation across all domains of the global energy sector. A vast wave of reinvention is transforming every part of the modern energy system, from long-distance transmission to energy storage, from residential heating to power grid control. The remarkable recent progress in advanced nuclear reactor designs is one of the most exciting ongoing developments in the energy world, given their potential to not only generate heat and power safely, reliably, and flexibly but also produce this energy without emitting carbon pollution.
The term “advanced nuclear reactor” refers to a broad category of fission reactor designs that boast considerable improvements relative to current-generation nuclear technologies. These innovations can result in a high degree of inherent or passive safety, high reliability, improved efficiency, lower costs, more complete utilization of nuclear fuel, lower generation of waste, enhanced resistance to nuclear proliferation, and versatile applicability for the production of non-electric co-products like hydrogen, high-quality waste heat, and desalinated water. Some of these improvements can be linked. For example, smaller reactor designs offer safety benefits thanks to smaller fuel loads and more efficient cooling characteristics while also reducing costs by facilitating factory assembly and transportation to the project site. Other factors, such as the ability to safeguard spent fuel, vary based on the specific design. This report considers the category of advanced nuclear reactors to include smaller next-generation light-water fission reactors in addition to non-light-water fission reactors.
While numerous forms of clean electricity generation like solar, wind, and hydroelectricity are already widely deployable today, nuclear power offers a number of unique advantages that help complement and support the deployment of other zero-carbon emissions technologies, thereby strongly incentivizing future advanced nuclear projects.
First, nuclear reactors produce substantially more energy relative to their land footprint than solar and wind projects, which require over 30x and 100x the land area for the same nameplate generating capacity. With nationwide land requirements for renewable energy sources under some modeled future scenarios exceeding the area of West Virginia, land use and siting constraints may increasingly favor nuclear projects. Nuclear facilities can also be located more flexibly than renewable projects that depend on sun and wind conditions. Combined with the potential ability of new microreactors and small reactors to match the needs of a range of customers from rural and island communities to remote industrial sites like mines, advanced reactors have the potential to serve a more diverse set of markets than previous generations of large, centralized nuclear power stations. Nuclear deployments, if proactively planned, could thus help reduce system-wide costs for a clean energy transition by limiting excess transmission and new grid infrastructure that extensive wind and solar installations would otherwise require.
Furthermore, many advanced reactors under development are being designed for high compatibility with variable renewable generation, with desirable operating characteristics such as accelerated ramping of generation to balance fluctuations in renewable output and even thermal energy storage capabilities. Academic research suggests that pairing reliable, clean, firm electricity from sources like nuclear power with variable renewable generation makes planned transitions to clean energy systems more affordable.
As an additional utility beyond that provided by solar and wind resources, nuclear reactors also generate useful heat and steam that can be utilized in industrial processes like desalination and hydrogen electrolysis. In comparison to traditional nuclear reactors, some advanced reactor designs can produce hotter outlet steam that can enable higher-efficiency hydrogen production from high-temperature water splitting and replace fossil fuel combustion in a wider range of industrial activities like petrochemical and cement manufacturing. Advanced reactors thus possess versatile nonelectric applications in industries well beyond the power sector.
Given the clear potential advanced nuclear technology offers, it is not surprising that numerous research groups and prospective vendors around the world are developing a range of advanced designs, with particular progress in China, Russia, the United States, Canada, and the United Kingdom. In a pair of 2020 reports, the International Atomic Energy Agency counted over 70 small advanced reactor designs and 12 advanced large water-cooled reactor designs worldwide in various stages of development. Notable small modular nuclear reactors to enter commercial operation include the two small pressurized-water 35MW KLT-40S units aboard the Russian floating nuclear power plant Akademic Lomonosov, officially commissioned in May 2020. In December 2021, the China National Nuclear Corporation commenced regular operation of the HTR-PM high-temperature gas-cooled pebble-bed reactor, a 200 megawatt-electric (MWe) unit sited in China’s Shandong province.
There are no advanced nuclear energy projects currently in operation or under construction in the United States, but several reactor designs are at various stages of licensing and regulatory approval. A growing number of initial planned projects have already been announced, including but not limited to the BWRX-300 project near Oak Ridge, Tennessee, and the Natrium project in Kemmerer, Wyoming. Estimated project completion dates typically lie in the late 2020s and early 2030s, with plans to expand deployment further upon successful demonstration.
Successful demonstrations and deployments of new advanced designs will rapidly accelerate progress towards widespread commercialization. Building a vibrant advanced nuclear industry in the United States will require sharp strategic planning. Industry stakeholders will need to proactively navigate policy obstacles, cultivate adequate fuel supply and manufacturing capacity, ensure sufficient capital investment, skillfully manage financial risks, and more. The process of recruiting, training, retaining, and growing the talent pool necessary to license, fabricate, assemble, operate and maintain next-generation reactors is both a challenge and opportunity for the advanced reactor sector.
Well-designed federal policies and programs can significantly facilitate the industry’s efforts on every front. At a time of deep division in Congress and the federal government, the deployment of new nuclear energy alternatives to fossil energy is one of the very few national priorities to enjoy appreciable bipartisan support. Consensus on Capitol Hill signals the urgency with which constituents expect their representatives and the Biden administration to reduce carbon emissions in the energy sector. To efficiently foster a domestic advanced nuclear sector, it will be essential for policymakers, investors, and industry to act in close cooperation with one another according to a rigorous, strategic plan for deployment.
This report seeks to describe, for policymakers and financiers, the key components that any successful advanced nuclear deployment plan will have to include. Using updated assumptions for advanced nuclear costs and cost improvements over time, this study modeled the evolution of the US power grid over the next three decades using the Weather-Informed energy Systems: for design, operations and markets-Planning Version (WIS:dom-P®) optimization model, developed by Vibrant Clean Energy, LLC (VCE®). The model evaluates the full energy system and designs a future net-zero CO2 power sector that meets demand at minimal cost.
This analysis finds considerable potential for advanced reactors to support future US electricity needs and climate progress. Inclusion of advanced nuclear designs among the available technology options for a clean energy transition leads to large-scale advanced reactor deployment as part of a least-cost pathway to a clean electricity future. However, the degree to which the United States can successfully develop an advanced nuclear energy sector over the next 15 years will crucially depend upon mobilizing sufficient capital investment and public policy support starting immediately from the present day.
The following chapters explain the high potential importance of advanced nuclear power to the future US energy sector and propose key investments, strategies, and policies that will help unlock the full potential of this emerging, promising, and powerful set of clean energy technologies.
Evaluating the Role of Technological Learning and Deployment for a Net-Zero Electricity Grid
1. Emerging Technologies and Technological Learning
New technologies are often expensive when first introduced, becoming increasingly cheaper, more affordable, and more competitive with time as more capacity is deployed. Indeed, one of the most encouraging clean energy triumphs of the past decade has been the rapid and dramatic reduction in the price of solar photovoltaic modules, wind turbines, and lithium-ion batteries. The cost of 1 megawatt-hour (MWh) of solar electricity or lithium-ion battery storage capacity has fallen by around 85 percent from 2010 to 2022. These shifts are already catalyzing fundamental changes in the electricity sector in many parts of the world. Worldwide solar capacity reached 707.5 gigawatts (GW) in 2020, growing 18 times relative to installed capacity at the start of the decade, while global wind capacity quadrupled over the same period.
However, full decarbonization of the power sector both globally and in the United States remains a long-term future goal that will require proactive planning and decades to achieve. A range of current and emerging clean energy sources will collectively work together in the effort to meet this objective. Clean, firm generating technologies like advanced nuclear reactors will complement wind and solar capacity, compensating for variable renewable electricity production, meeting demand in regions with low wind and solar resource potential, and alleviating other constraints such as land availability and transmission costs.
Advanced nuclear technologies will also likely experience cost improvements over the next decades as developers move from their first demonstration projects to successively larger waves of deployment. However, not all technologies necessarily become more affordable at the same rate. Researchers have long understood that, over time, costs evolve differently for different technologies based on numerous factors, and have employed concepts like learning rates and learning curves to analyze such trends.
A learning rate reflects the rate at which a technology achieves cost improvements as it becomes more established in the market. A learning rate of 5 percent for a generation technology, for instance, means that the capital cost of that technology decreases over time by 5 percent for every doubling of installed capacity. Over time, the falling cost of the technology can be graphed along a curve, referred to as a learning curve.
Technological learning rates for nuclear power have been studied extensively, with various estimates and results. A 2016 study calculated the overnight cost curve of nuclear power across time in seven countries. The costs of commercial nuclear plants increased dramatically from approximately 1965 to 1975 in the United States, and increased slightly in France from 1970 to 1990.
Whereas the increasing affordability of technologies like batteries and semiconductors has demonstrated the success of large-scale learning-by-doing, recent efforts to reduce nuclear energy costs through mass deployment have encountered setbacks. In recent decades, prominent conventional nuclear projects in Western countries like Olkiluoto-3 in Finland and Vogtle units 3 and 4 in the United States have experienced marked cost overruns and construction delays. A handful of earlier studies of the increasing nuclear construction costs in the United States and France even alleged negative learning rates for traditional nuclear plants. ,
However, escalating conventional nuclear costs may reflect poor planning, engineering, and policy frameworks rather than inherent technological factors. A comprehensive global analysis of nuclear power plant construction costs for plants built around the world between 1954 and 2015 (from which the increased costs mentioned above are drawn) found that many national nuclear programs exhibited considerable cost improvements during the early phase of nuclear deployment. Other nations like Japan experienced very limited cost escalation, with nuclear builds in South Korea even achieving cost declines. While costs of nuclear projects in China are unclear, the Chinese government has pursued an aggressive buildout of conventional nuclear over recent decades, with the majority of builds completed after 2000 requiring between five and seven years for construction.
When researchers reviewed the literature on nuclear learning rates they found estimates of learning rates that varied from -49 percent (rising costs with time) over cumulative national nuclear deployment, to +11 percent (falling costs over time) for projects constructed in series by the same firm. , All six studies that included a specification to control for the same construction firm found a positive learning rate using US data, indicating that costs of reactors from the same firm may have declined from learning, internal to the firm, even as national nuclear costs escalated. This may provide evidence that serialized and standardized construction of nuclear plants, as is often proposed by advanced nuclear developers, could lead to significant positive learning and cost declines over time.
With a new generation of nuclear technologies now poised for deployment, historical nuclear cost patterns may no longer serve as an appropriate basis for projecting the future competitiveness of nuclear power. Multiple studies have used bottom-up models to estimate potential learning rates for advanced nuclear technologies. For small modular reactors (SMRs), five of the six studies estimated positive learning rates, ranging from 3.4 percent to 16 percent across studies and scenarios. In 2022, Stewart and Shirvan estimated a 16 percent learning rate using a bottom-up model that estimates costs of factory build, labor, and materials, in addition to deriving estimates from the cost record of relevant technologies like natural gas turbines, wind turbines, and small airplanes. Their study concludes that while first-of-a-kind (FOAK) costs may be significantly higher than conventional nuclear costs, the accelerated learning that is enabled by modularization and novel technology could result in significant cost declines. Furthermore, by reducing the total hours of labor required, advanced reactors could avoid the risk of cost overruns that have been associated with labor-intensive megaprojects. Reducing the risk associated with project size and labor requirements could make advanced nuclear a more attractive investment for utilities, policymakers, and financiers.
1.1 Prospects of Advanced Nuclear for Achieving High Learning
In technical aspects, advanced reactors represent a sufficient departure from traditional light-water reactors of previous generations that they may exhibit improved learning rates. At the same time, advanced nuclear designs may be able to achieve a relatively more rapid pace of commercial deployment, enabling these technologies to benefit more quickly from cost reductions.
Broadly, the advanced nuclear industry is seeking to shift nuclear manufacturing and construction into a process more akin to building “airplanes not airports”. More modular reactor units can be more easily factory-manufactured, without as extensive requirements for expensive heavy forging of components. A smaller reactor will also reduce construction time, while enabling more standardization and simplification in balance-of-plant infrastructure. Some modular advanced nuclear designs are intended to be manufactured in a central facility, then transported to the site of installation in a “hub and spoke” production model. Units could even be returned whole to the manufacturer following retirement, simplifying the decommissioning process and reducing end-of-life costs. Smaller designs also allow advanced reactor vendors to scale projects modularly to compete in a wider array of applications, potentially increasing demand and driving faster rates of deployment and learning.
Aside from the supply chain advantages offered by moving towards reactor units a fraction the size of conventional light-water nuclear plants, advanced reactor concepts also possess inherent characteristics that may drive lower costs. For instance, advanced designs that are capable of passively self-cooling for weeks in the event of a loss of onsite power may require less backup generating infrastructure. New nuclear technologies that operate at normal atmospheric pressure may not require the specialized reactor vessels and sophisticated systems of pumps and valves needed to maintain higher pressures in, say, a traditional pressurized water reactor.
In general, modular advanced reactors feature much more streamlined design principles, with fewer complex parts and a reduced need to maintain multiple, intricate, redundant systems. Stewart and Shirvan’s study concluded that small reactor designs in particular could benefit from five factors that could lead to accelerated learning and reduced costs: 1) deployment of many units at the same site, 2) serialized factory manufacturing, 3) shortened construction time, 4) design simplification of plants, and 5) increased flexibility of deployment to maximize system value.
A 2018 study concluded that the costs of materials like concrete, steel, and nuclear fuel have not been major cost drivers of nuclear plants; instead, design, labor, and project management have been significant determinants of construction costs. In particular, high-cost plants often: do not have completed designs at the time of construction start, experience significant regulatory interventions during construction, have a FOAK design, encounter litigation between project participants, have a long construction schedule, and have high labor rates and high labor hours invested. Since many of these factors could potentially be addressed by serialized deployment of advanced nuclear technologies, we attempt to model the potential learning achievable by advanced nuclear deployment in our modeling analysis.
1.2 Approach for Modeling Advanced Nuclear Costs and Potential for Learning
This study assesses the scale of public policy support and capital deployment needed to drive the successful deployment of advanced nuclear reactors at scale in the United States, and provides insight into a least-cost energy system that incorporates advanced nuclear power to achieve a net-zero power sector.
Recognizing the uncertainties around future learning rates and construction costs of advanced nuclear, we use a range of assumptions for learning rates and FOAK costs that are grounded in the literature. The four scenarios in the modeling results that follow are distinguished by “high” vs. “low” learning rates and FOAK cost inputs (Table 1-1). For a detailed summary of study assumptions and methods, see Appendices A and B, respectively.
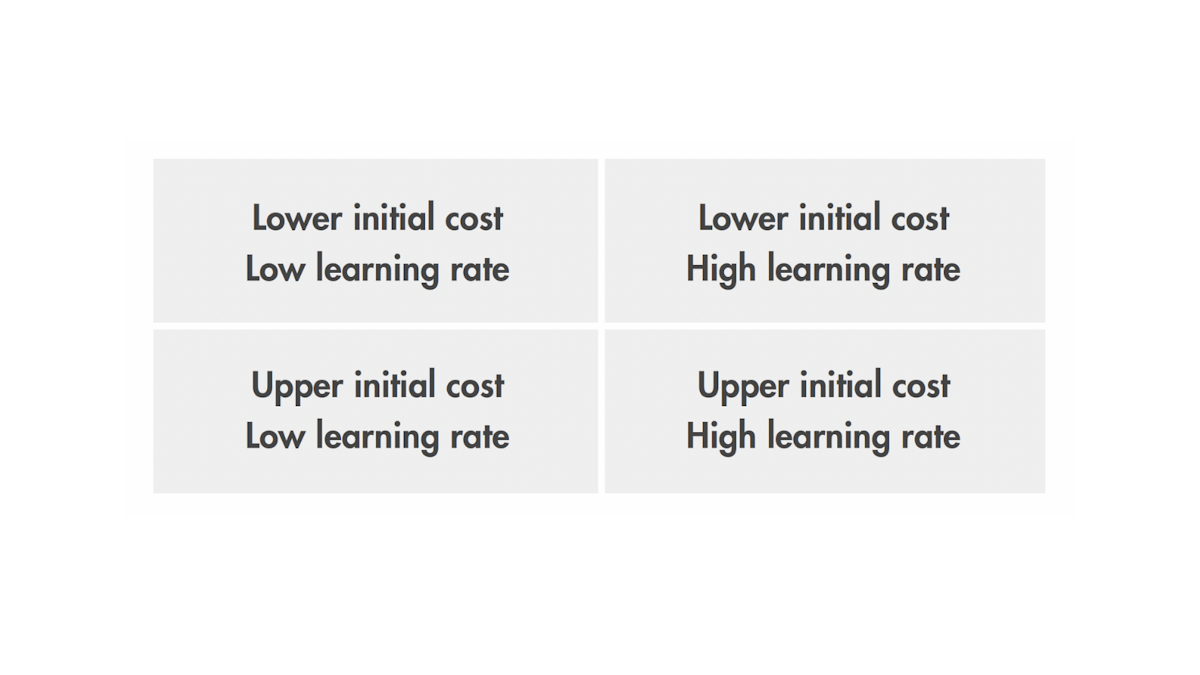
Cost Reduction Due to Learning
To reflect learning over time, other studies have generally incorporated pre-determined cost reductions over time for energy technologies like solar photovoltaic (PV), wind, nuclear, natural gas with carbon capture and storage (CCS), and other clean energy sources. Improving over this prescribed approach, our study utilized a dynamic endogenous approach to modeling advanced nuclear reactor deployment costs. This approach updates the capital costs of advanced nuclear technologies based on a scenario-specific learning rate to reflect improvements as the model independently deploys reactors over time. Further discussion of learning calculations is included in the methods section in Appendix C.
An imposed capital cost floor for all three advanced nuclear technologies was set at $1,800 per kilowatt-electric (kWe). In addition, we initialize learning in the model by hard-coding a set of advanced nuclear demonstration projects already announced, funded, or otherwise planned. The projects include demonstrations of all technology types, and the model is restricted from building any non-demonstration advanced nuclear projects before the end of 2028.
Learning Rate Selection for Scenario Development
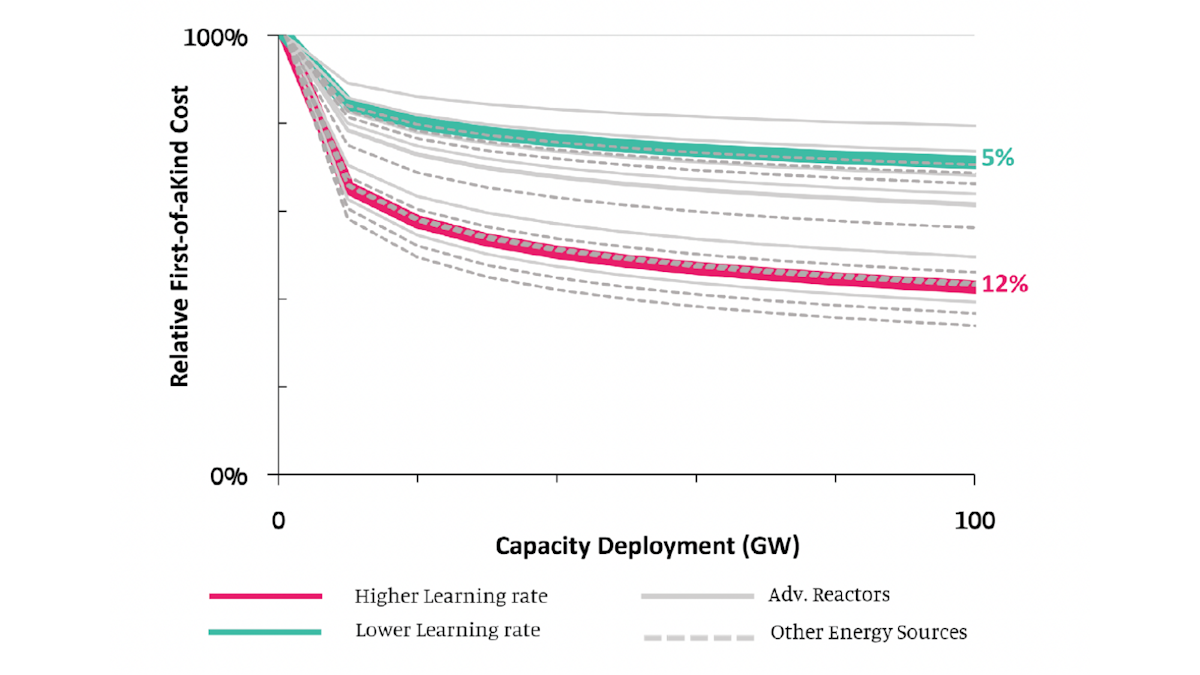
This study uses learning rates of 5 percent (Low Learning) and 12 percent (High Learning) based on the estimates of advanced nuclear learning discussed above to effectively provide bounds on the potential impact of learning (Figure 1-1). For comparison to other energy technologies (see Appendix B), a learning rate of 5 percent approximates the empirical learning rate of coal-fired power plants, while a learning rate of 12 percent approximates the empirical learning rate of wind power installations.
Costs for First-of-a-Kind Projects
Estimates of FOAK capital costs for advanced nuclear technologies were collected from the academic literature, national laboratory studies, and proprietary private sector estimates. For the purposes of this study, we examined three technological categories of advanced nuclear reactors: light-water SMRs, high-temperature gas-cooled reactors (HTGRs), and advanced reactors with thermal energy storage (ARTESs). These three categories of advanced reactors are not comprehensive, but reflect many of the design types slated for deployment over the next decade in the United States. Input values are derived from multiple sources to represent the technology class and do not reflect a single design. As used in this report, the ARTES design has many of the characteristics of liquid-metal or molten-salt fast reactors, but thermal storage can be used with most nuclear reactor types.
Certain fixed and variable costs—which remain static throughout the duration of the model—were also adjusted across the two Upper Cost and Lower Cost scenarios. Importantly, conventional nuclear does not undergo endogenous learning in this model as it is a well-established technology. Instead, in Lower Cost scenarios, an assumed lower bound capital cost for conventional nuclear plants is held static over the course of the model. Meanwhile, in Upper Cost scenarios, a prescribed cost reduction for conventional nuclear power is assumed in accordance with the National Renewable Energy Laboratory (NREL) moderate Annual Technology Baseline (ATB) projection. NREL ATB 2021 is the most recent version available and is used in this study. Table 1-2 summarizes the input cost assumptions and assumed learning rate regimes for advanced and conventional nuclear energy in all four modeled scenarios. Further details regarding model settings and assumptions appear in Appendix A.
Nuclear Technology Input and Learning Assumptions Across Scenarios
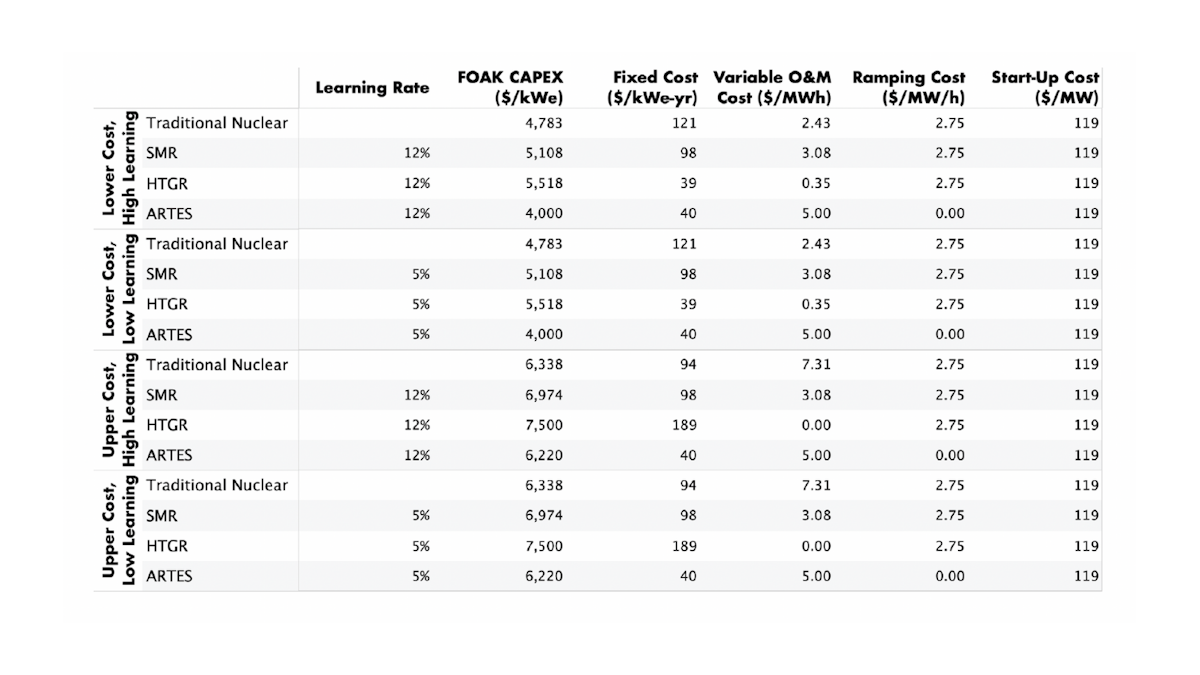
1.3 Operational Characteristics
In addition to initializing FOAK capital costs and gathering fixed and variable nuclear cost assumptions, this study applies technology-specific operational characteristics that affect grid service capabilities, siting, and more. Table 1-3 presents these input characteristics, including heat rate, template reactor capacity size, maximum power output to the grid, minimum operational power output, maximum up and down ramping capabilities, liters of water consumption per kilowatt-hour (kWh) of electric energy generated, and, for ARTES, the electric energy equivalent of thermal storage capacity and roundtrip thermal storage energy efficiency. Maximum output is considered to be the same as reactor capacity except for ARTES.
Nuclear Technology Input and Learning Assumptions Across Scenarios
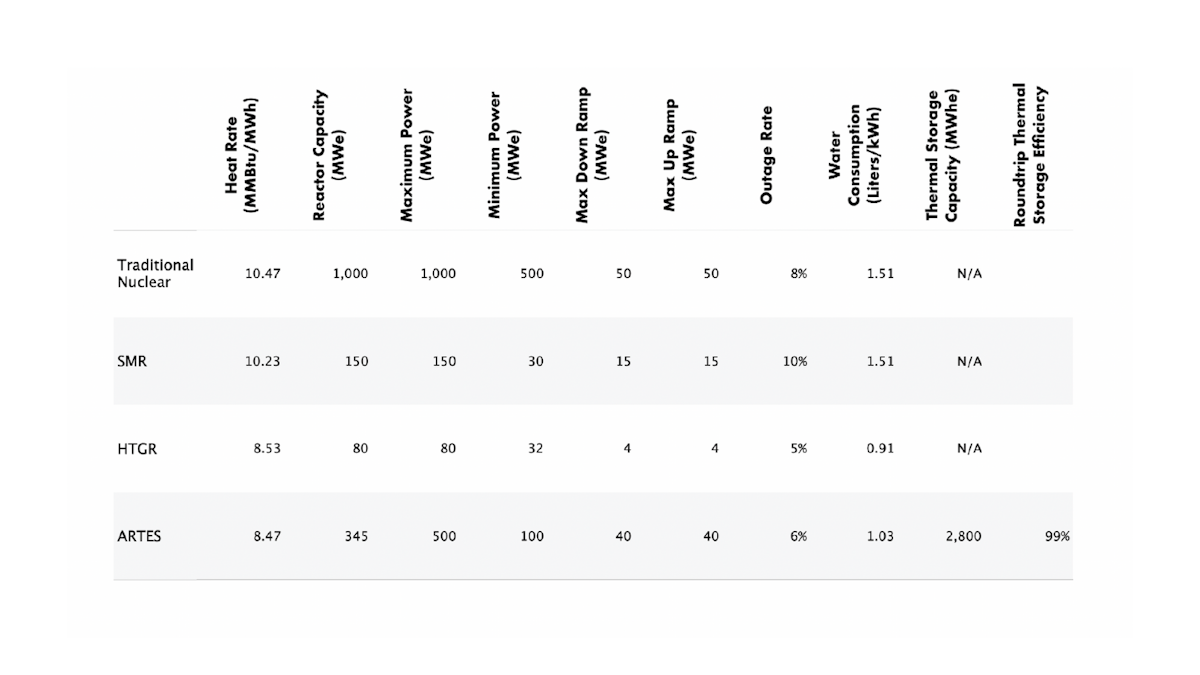
These detailed operational characteristics for advanced nuclear reactors alongside the above mentioned input cost and learning assumptions served as inputs to the WIS:dom-P model to investigate how the United States electricity sector changes in order to achieve decarbonization by 2050 at the lowest feasible cost for the utility-scale power sector. These operational data and bounding cost and learning assumptions reflect the latest literature and do not inherently favor advanced nuclear technologies over other clean technology options. At the same time, this approach represents a progression relative to other energy system studies that typically only rely on specifications and future cost projections for large light-water conventional reactors to model nuclear power.
Establishing a cautiously bounded playing field for emerging nuclear technologies allows the range of roles that advanced nuclear power might play in tomorrow’s clean electricity grid to be fairly assessed. The next section summarizes this modeling study’s key findings and discusses their implications for the future US energy system.
2. A National Clean Electricity Grid by 2050
This study uses multiple input scenarios to model a future electricity system that reaches 99 percent decarbonization by 2050. The multiple input scenarios result in a range of energy source mix and structure.
2.1 Power Sector Mix and Structure
Capacity Expansion Modeling Results
By 2050, the US power sector landscape primarily consists of renewable and nuclear technologies in these model runs (Figures 2-1 and 2-2). Both installed generation capacity and total generation grow considerably in the next three decades over the course of the four scenarios. Installed capacity more than doubles, increasing to around 2,500 to 3,000 GWe by 2050. Total nationwide generation grows from 3,900 TWh in model year 2020 to 7,400 TWh in 2050.
Installed Capacity
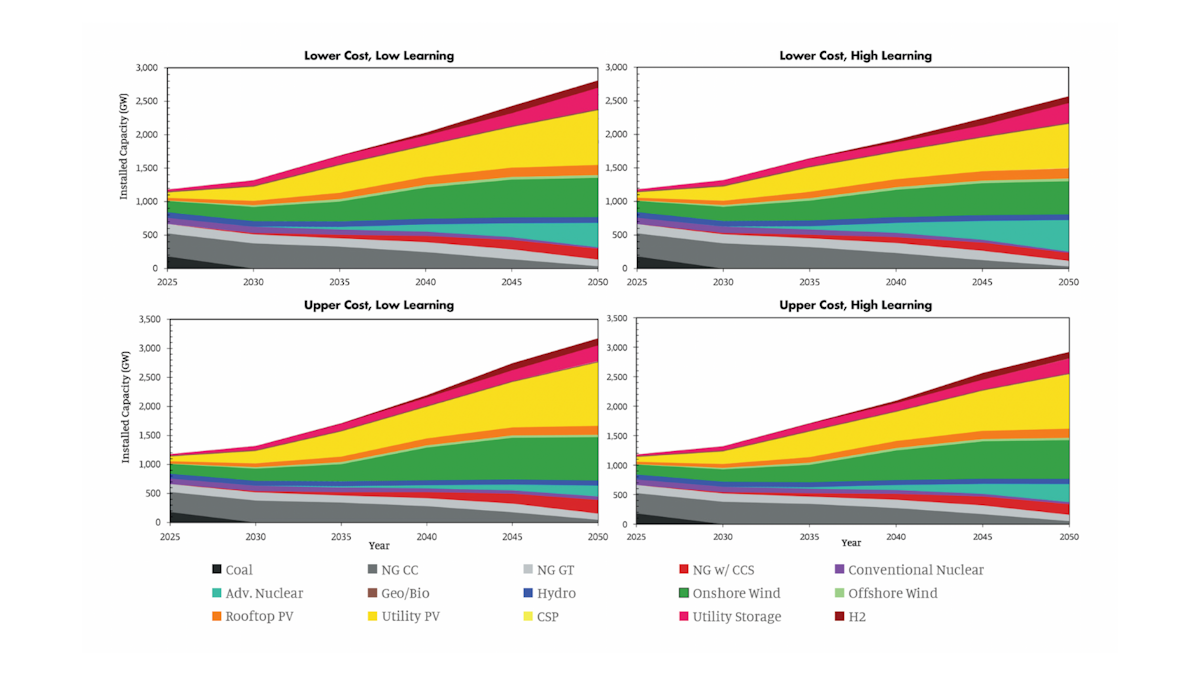
Renewable energy grows across all scenarios, dominated by onshore wind and utility-scale solar photovoltaic (UPV) installations. The shift to low-carbon technologies results in the retirement of coal generation by 2030 in all models, as well as the gradual diminution of natural gas generation and capacity. Ultimately, only natural gas combined cycle plants with carbon capture and storage (NGCC+CCS) are in operation by 2050.
Advanced nuclear technologies increase across all scenarios, with significant deployment beginning in 2035. By 2050, advanced nuclear power annually generates a comparable amount of electricity to utility-scale solar farms in the Upper Cost scenarios. In the scenarios with lower initial advanced nuclear capital costs, advanced nuclear plants produce a comparable amount of national electricity as utility-scale solar and onshore wind installations combined.
Utility-scale electricity storage and distributed rooftop solar PV capacity both increase steadily throughout the next three decades. Meanwhile, the proportion of US electricity generation from hydroelectric stations declines modestly. Traditional nuclear power also plays a progressively smaller role in the energy mix through 2050 due to high capital and operational costs and plant retirements.
Electricity Generation
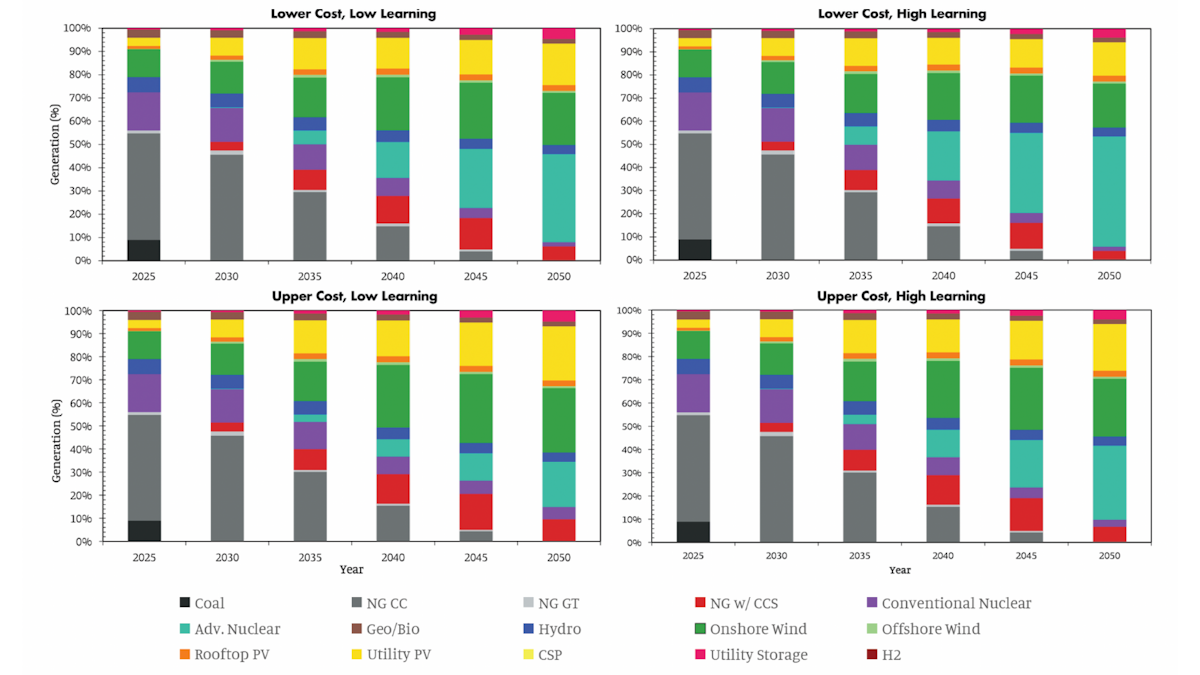
Only late in the Upper Cost, Low Learning scenario does conventional nuclear exhibit a total net increase in nationwide capacity, expanding from 57 GWe in 2045 to 62 GWe in 2050. This deployment is driven by the combination of higher capital costs for advanced nuclear (this scenario represents the most conservative bounding scenario for advanced nuclear capital costs and cost reductions) and the constraining requirement that the model decarbonizes by 2050. In the two Upper Cost scenarios, especially in the Upper Cost, Low Learning scenario, traditional nuclear sees an uptick in new deployment in several states.
Geographic Distribution of Capacity
The WIS:dom-P model deploys new power sector infrastructure at specific geographic sites with county-level granularity at minimum, based on factors such as competing land uses, renewable resource quality, cooling water availability, and existing transmission networks. Some differences in siting decisions occur between the four scenarios due to differences in advanced nuclear capital costs and cost improvements over time. Overall, however, patterns of capacity installation over the next three decades are geographically similar for all scenarios.
Across the four model scenarios, by 2050, a cumulative land area of 17,500 to 28,800 square kilometers (1.75-2.88 million hectares) nationwide is directly occupied by wind turbines and utility-scale solar projects, as well as associated roads and infrastructure. This direct land usage ranges between the combined area of Connecticut and Rhode Island and the total area of Massachusetts. When indirect land use including the land surface surrounding solar modules and wind turbines is taken into account, a total cumulative land area of 182,000 to 277,000 square kilometers (18.2 - 27.7 million hectares) nationwide is occupied by wind and solar farms.
New advanced reactor projects are distributed broadly across the continental United States by 2050 (Figure 2-3). Large facilities are generally concentrated near major load centers such as across the eastern coastal states between Washington, DC, and Boston, the Great Lakes region, and the Pacific Northwest. Sizable advanced nuclear power stations are also situated near Houston, Los Angeles, Las Vegas, and Phoenix.
Utility-scale solar and onshore wind farms are numerous throughout the country. Particularly sizable concentrations of utility solar PV generation are deployed in California and Texas and throughout much of the midwest, southeast, and mid-Atlantic regions. Onshore wind capacity is prevalent across the Great Plains, the Midwest, and Texas. Moderate amounts of offshore wind capacity are installed along the Atlantic coast between North Carolina and Massachusetts.
Installed Capacity in 2050
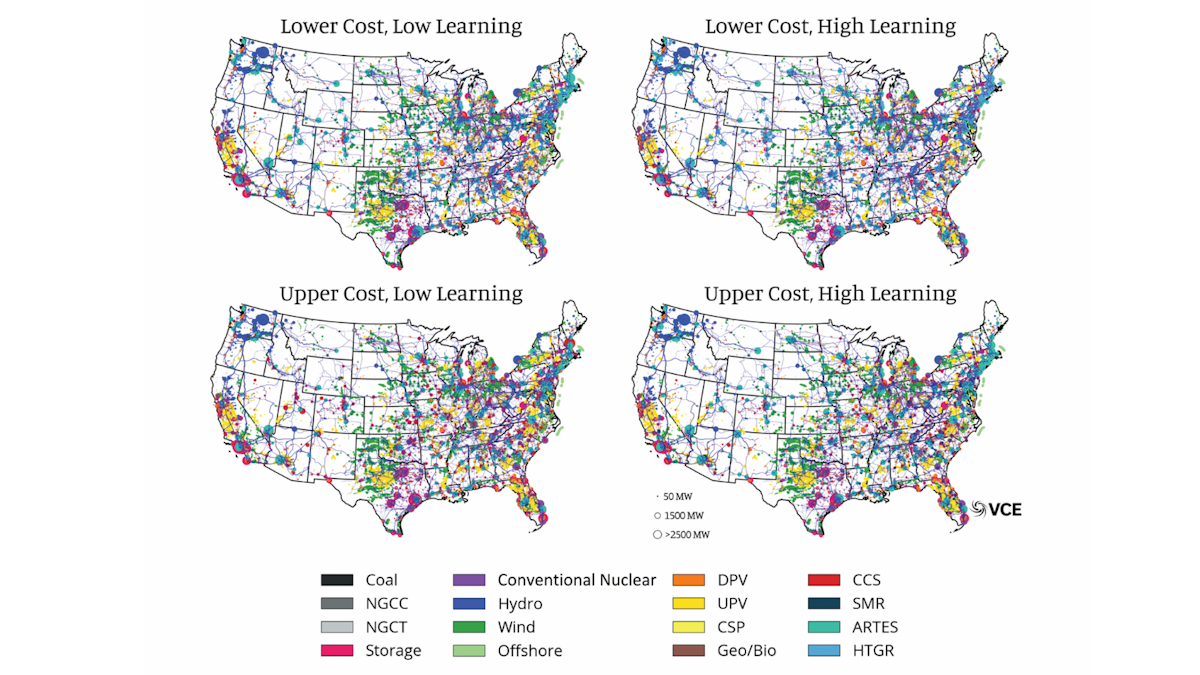
Interestingly, across all scenarios, traditional nuclear sees a modest resurgence of new deployment in Florida between 2040 and 2050. The Pacific Northwest continues to generate a large proportion of regional electricity from hydropower. Finally, large utility-scale energy storage facilities follow a siting pattern somewhat similar to advanced nuclear plants, with sizable deployments in urban areas of California, Texas, Florida, and the Eastern seaboard.
Load Profiles and Grid Dispatch
A distinguishing aspect of this study and a powerful feature of the WIS:dom-P model is its exceptional level of temporal detail in assessing grid dispatch, at up to five-minute intervals. Importantly, this enhanced temporal resolution provides additional advantages compared to modeling dispatch at a coarser hourly interval. This feature allows for a high confidence that available grid supply consistently meets demand and ensures continued reliability even as variable renewable energy generation expands.
In general, advanced nuclear designs can ramp generation output at rates faster than traditional nuclear technology. ARTES technologies possess the particularly attractive ability to store energy in thermal energy storage systems and increase power output later, allowing for effective load and price following while maintaining very high reactor capacity factors. The flexibility of advanced nuclear manifests in sharp ramping to meet peaks and valleys in generation from low-cost, fuel-saving variable renewables. Figure 2-4 illustrates this ramping behavior within a net-zero electricity grid for all four scenarios during a simulated summer in 2050.
Example Summer Grid Dispatch in 2050
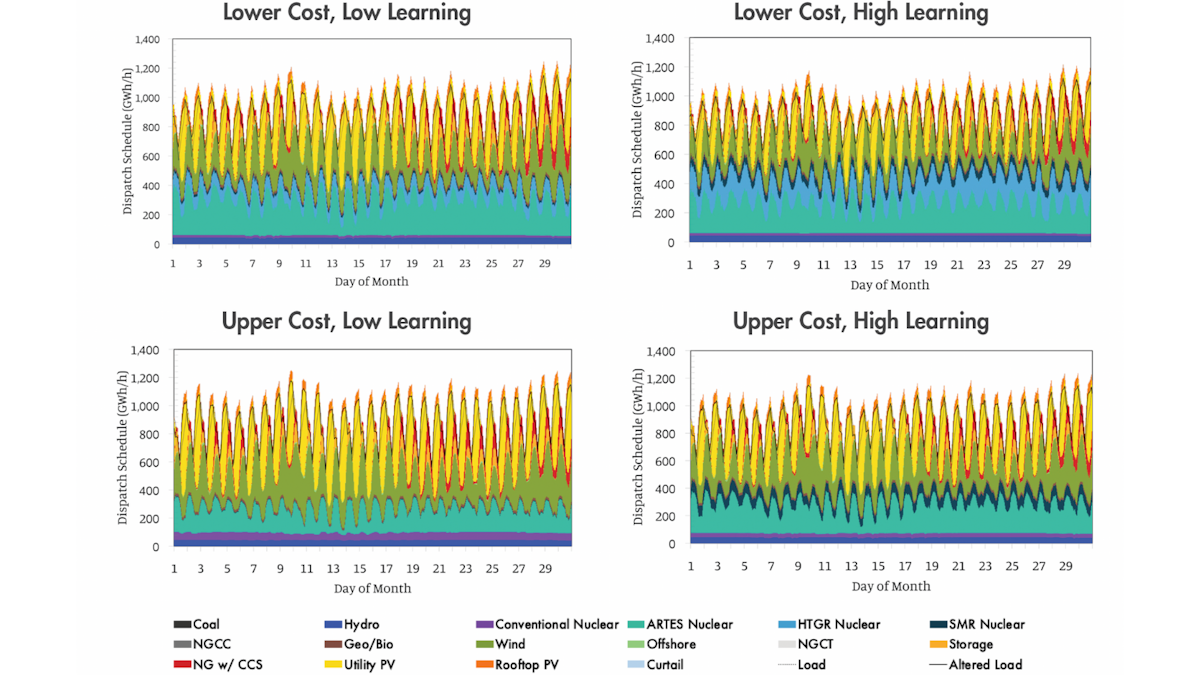
2.2 Advanced Nuclear Deployment
Advanced nuclear power plays a prominent role in achieving a net-zero electricity grid, with 185 to 469 GWe of advanced nuclear capacity providing around 20 percent to 48 percent of total electricity generation in 2050 (Table 2-1 and Table 2-2). Since this model features endogenous learning, advanced nuclear technology deployment and cost reductions go hand in hand. As the model independently elects to build advanced nuclear alongside other clean energy technologies as part of a least-cost energy system solution, nuclear technology overnight costs decline over time due to endogenous learning. Using accurate advanced nuclear operational characteristics and employing a bounding analysis of four different sets of initial overnight cost assumptions and learning rates, the WIS:dom-P model deploys a large amount of advanced nuclear capacity throughout the contiguous United States over the next three decades.
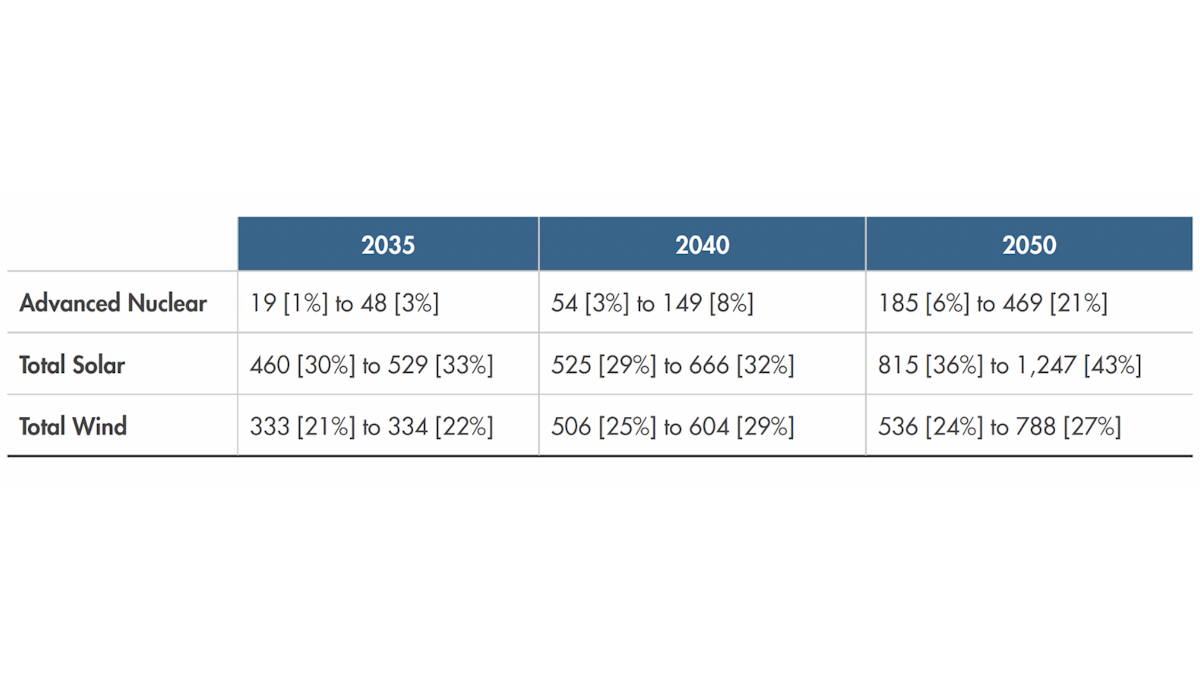
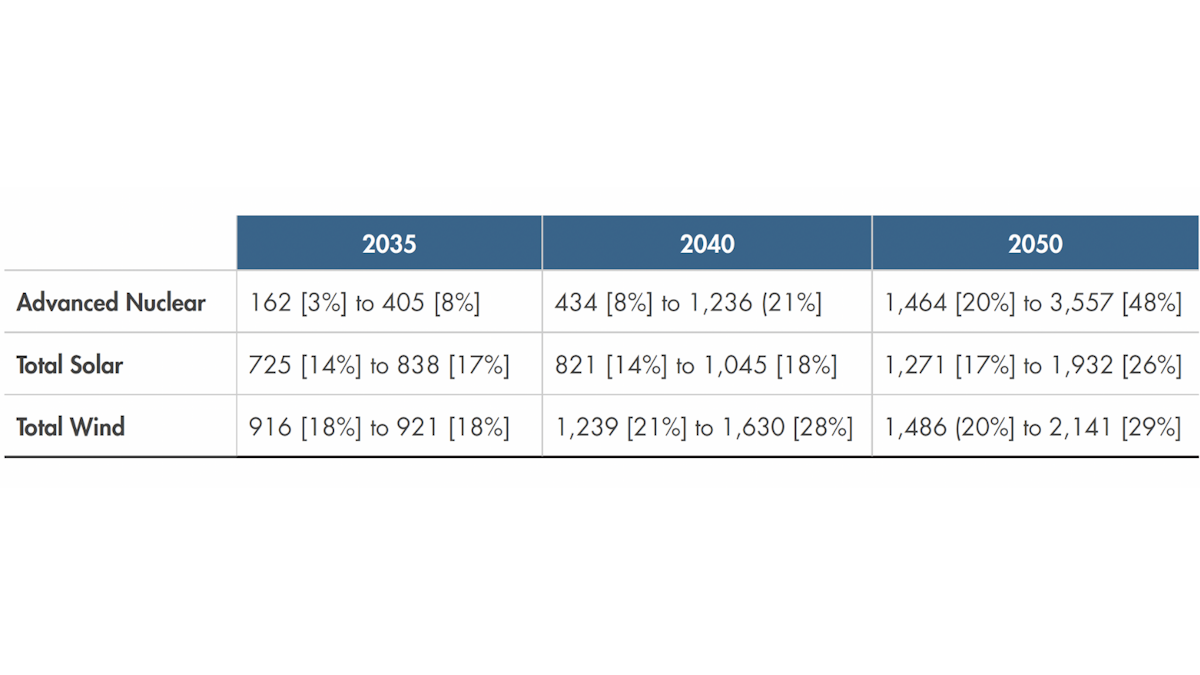
This result corresponds to the following number cumulative total of advanced nuclear reactor units across the three modeled technologies and reactor sizes, shown in Table 2-3.
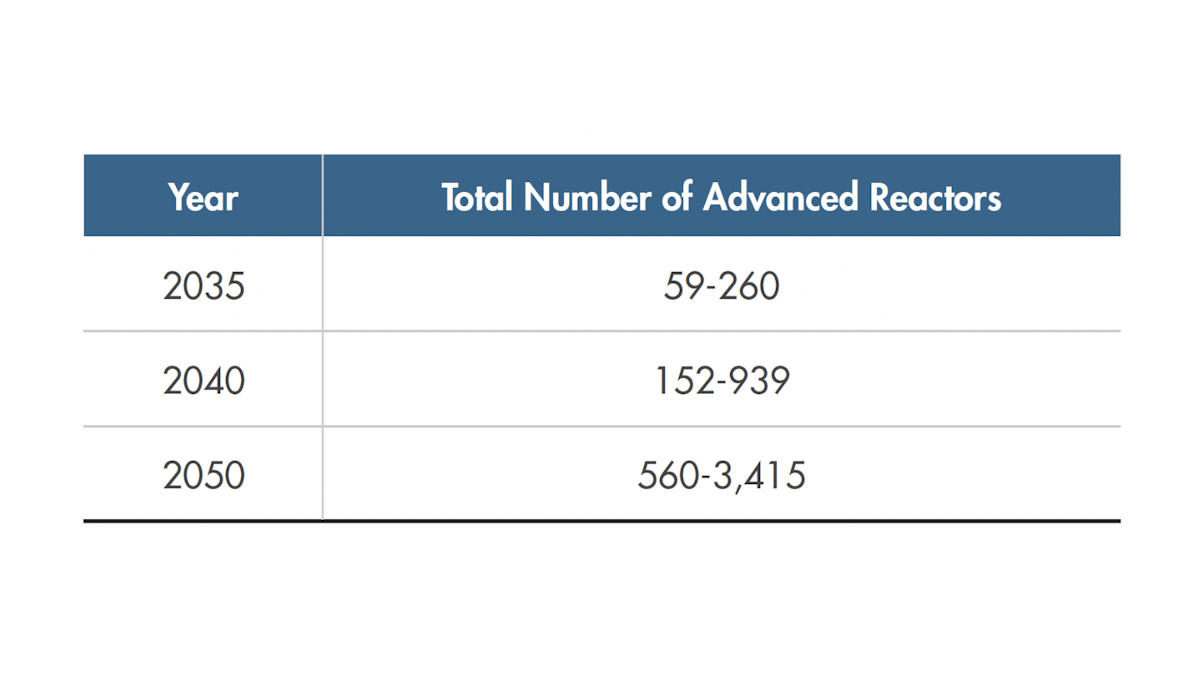
Unsurprisingly, the highest values for capacity and generation occur under the Lower Cost, High Learning scenario in which advanced reactor designs are initially demonstrated at a more affordable cost and experience faster learning by doing, eventually driving substantial cost reductions over time.
Both traditional and advanced nuclear power compete against NGCC+CCS in the model in terms of capacity expansion and energy dispatch, yielding insights into capacity factors and levelized costs of energy for different clean firm power resources. The advanced nuclear power plants generally exhibit high capacity factors of >80 percent nationwide, indicating high utilization and good market competitiveness. Figure 2-5 shows temporal trends in total electricity generation and average capacity factor for different clean firm technologies that see significant deployment in the capacity expansion model.
Temporal Generation for Clean Firm Power Across Scenarios
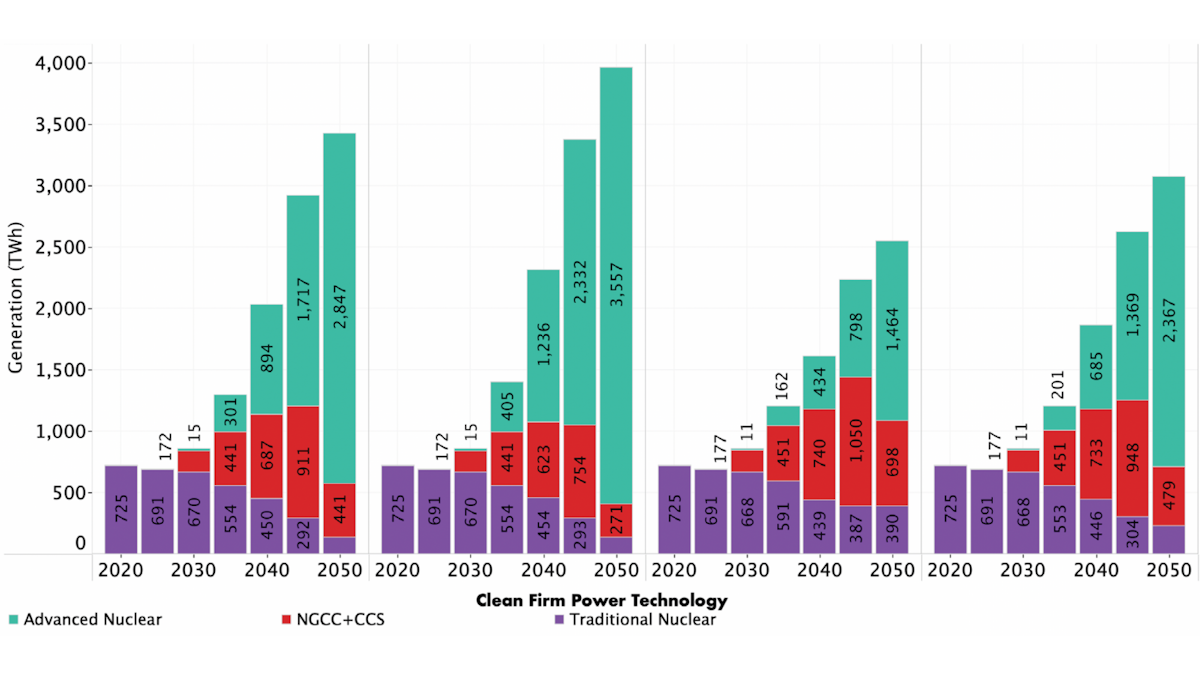
A notable conclusion from this study is that as the model approaches 2050, it utilizes NGCC+CCS less, primarily leveraging fossil gas generation with CCS for ancillary firming purposes as opposed to large-scale energy generation. In contrast, nuclear energy generation is generally used at a high capacity factor, outcompeting NGCC+CCS in the modeled electricity market.
Deployment and overnight cost reductions due to learning
Over the next 30 years, advanced nuclear overnight cost (OC) reductions from endogenous learning encourage further deployment and drive continued cost declines in the WIS:dom-P model. This virtuous cycle can be expressed as a function of the number of deployed reactors, as this study’s endogenous learning is calculated using cumulative capacity, normalized to technology-specific template reactor capacity sizes.
In particular, reactor capacity sizes are 1,000 MWe for traditional nuclear, 150 MWe for SMRs, 345 MWe for ARTES (with a capability to ramp up to 500 MWe for 5.6 hours at a time), and 80 MWe for HTGRs. The inclusion of thermal energy storage results in unique and more sophisticated modeling constraints and capabilities.
Traditional nuclear power does not experience endogenous learning in this study. Instead, in the two Lower Cost scenarios, a static $4,783/kWe OC is assumed; and in the two Upper Cost scenarios, we adopt the NREL moderate ATB cost projections. More information is available in the Appendices.
This study ultimately produces significant advanced nuclear deployment, driving substantial OC cost reductions. Assessing these OC reductions as a ratio of nth-of-a-kind (NOAK) to FOAK capital costs contributes valuable insights into how far advanced nuclear progresses down the learning curve in the simulations. Figure 2-6 illustrates this learning curve progress for the three modeled advanced nuclear technologies across the four FOAK and learning rate scenarios.
Advanced Nuclear Overnight Cost NOAK to FOAK Ratio Learning Curve Progress Across Scenarios
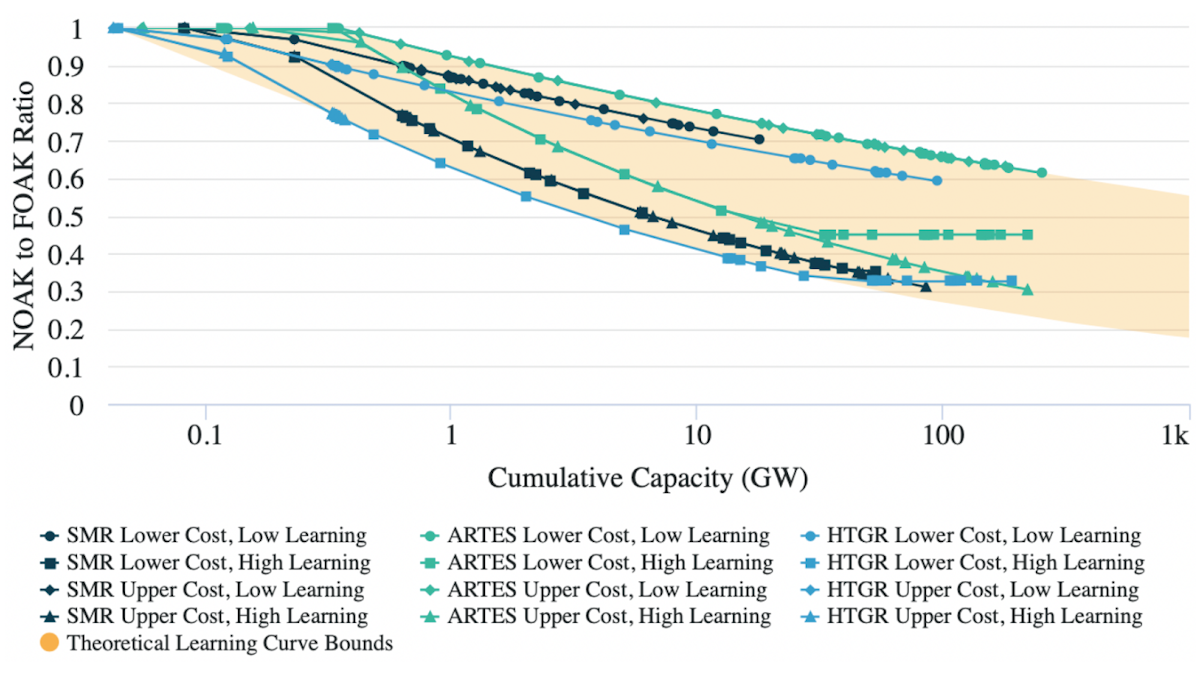
Figure 2-6 follows a classical learning curve where cost improvements continue with growing cumulative capacity deployment. However, an important feature of advanced nuclear technologies—and, in particular, those that employ standardized and modular manufacture—is that learning is better described as a function of reactor deployment, thereby benefiting technologies that can be sited more flexibly. The WIS:dom-P model used in this study attempts to deploy capacity in discrete increments as reactors, but also maintains the capability to flexibly site capacity deployment at a county-wide scale in increments that differ from reactor sizes.
Here, cumulative capacity deployment (GWe) and overnight cost ($/kWe) data in Figure 2-6 are exponentially interpolated to annual data from five-year cumulative data, where the incremental increase in each five-year period is annualized in years one through five. Exponential interpolation is used in favor of linear interpolation because this method is in line with the modeled five-year period cumulative capacity deployment data. Details on the exponential interpolation methods used for incremental annualized data are briefly discussed in the methods section in Appendix B.
2.3 Limitations
Ultimately, not even sophisticated national energy system models are capable of confidently predicting the clean energy future with certainty. As is the case for any study of this scale, the results described throughout this report are sensitive to numerous factors, from the range of assumptions considered to the technical and design constraints of the selected modeling approach. A number of the more important limitations are discussed in this section.
This report is confined to the continental United States and therefore does not account for advanced nuclear deployment that could benefit overall learning rates due to projects built in Alaska, Hawai’i, the US Territories, or internationally via nuclear export projects. This analysis also only considers advanced nuclear deployment in support of the US electricity sector and therefore does not directly account for nuclear deployment in service of non-electric markets, such as the market for high-heat applications. Advanced nuclear projects that help meet industrial process heat demand might, for example, accelerate the deployment of HTGRs beyond those deployed for the power sector alone.
This modeling study also does not address some market factors that could influence the competitiveness of advanced nuclear designs with one another and with other energy sources. In reality, one class of reactors may achieve a low FOAK cost while another category is built at a high FOAK cost. Additionally, advanced nuclear technologies are likely to undergo learning at different rates. Various market factors will determine the relative competitiveness of different technologies, from the availability of high-assay low-enriched uranium (HALEU) fuel for initial projects to the varying success of vendors in securing business deals and pre-orders, to the efficiency with which upstream manufacturing capabilities are established, and more.
These findings are likely sensitive to such considerations, but fully exploring this solution space would have required an order of magnitude higher number of scenarios. In addition, assigning high or low FOAK costs to different technologies effectively picks winners and losers at the model parameterization stage. Given such considerations, this study aims to provide a bounding analysis that can constrain the high and low range of future costs and deployment of advanced nuclear reactors as a group, based on a high-resolution capacity expansion model.
New future non-nuclear clean technologies may also emerge, changing the trajectory of the domestic clean energy buildout. For instance, enhanced geothermal energy could also see more widespread commercialization this decade, an outcome the WIS:dom-P model has not accounted for in the set of scenarios. The costs of offshore wind deployments might fall more rapidly than anticipated at the same time that offshore wind capabilities increase. Alternatively, new forms of cheap or long-duration energy storage could enable even wider deployment of wind, solar, and storage capacity beyond anticipated levels.
The future of the US energy system is complex and continually changing. There is no guarantee that advanced nuclear power will grow to the extent envisioned in these models. These results highlight how advanced reactors, when modeled realistically, have the potential to make a major contribution toward national energy production and progress in climate change mitigation.
Required Investment, Strategic Thinking About Early Deployment, and Versatile Applications
3. Capital Investment for Early Advanced Nuclear Deployment
In the previous chapter, the results highlight how advanced nuclear power can grow to become a large component of the future energy system in the United States even under pessimistic cost and learning assumptions. However, this outcome assumes sufficient availability of capital. The magnitude of capital investment implied by the deployment timeline across these model scenarios demonstrates the scale of future investment needed for a successful advanced nuclear sector. In today’s world, the complex processes of technology development and product commercialization are inextricably intertwined with government policy and market interactions.
3.1 Capital Investment
Capital formation is the process of acquiring the necessary pool of capital for an investment or project. That capital can be in the form of financed debt or equity. Capital costs are generally expressed as overnight costs (OC). Nuclear construction projects occur over multiple years, with capital expenditure required both before and throughout the construction process. This modeling study produced estimates of capital expenditure for advanced nuclear projects over the next 30 years across four scenarios. In Figure 3-1, we show capital costs annualized over an assumed three-year construction period, which is consistent with stated NOAK construction timelines for most advanced reactor developers.
Capital must be available to enable initial advanced reactor projects and develop the necessary supply chain. Critically, early investment in advanced nuclear energy drives early learning and catalyzes the development of supporting services like manufacturing, construction, fuel supply, and more. Early planning for and incorporation of firm, clean, dispatchable generating capacity like nuclear can reduce total system-wide power sector costs for decarbonization scenarios, relative to inefficient retroactive deployment in response to emerging system needs.
Realizing the potential of advanced nuclear energy to help power the United States energy transition could require cumulative capital formation for power plant construction on the order of $150 to $220 billion investment by 2035, growing to a total of $830 billion to $1.1 trillion by 2050. For context, the same range of capital investment is required to deploy utility-scale solar and onshore wind across the model scenarios.
Cumulative Annualized Capital
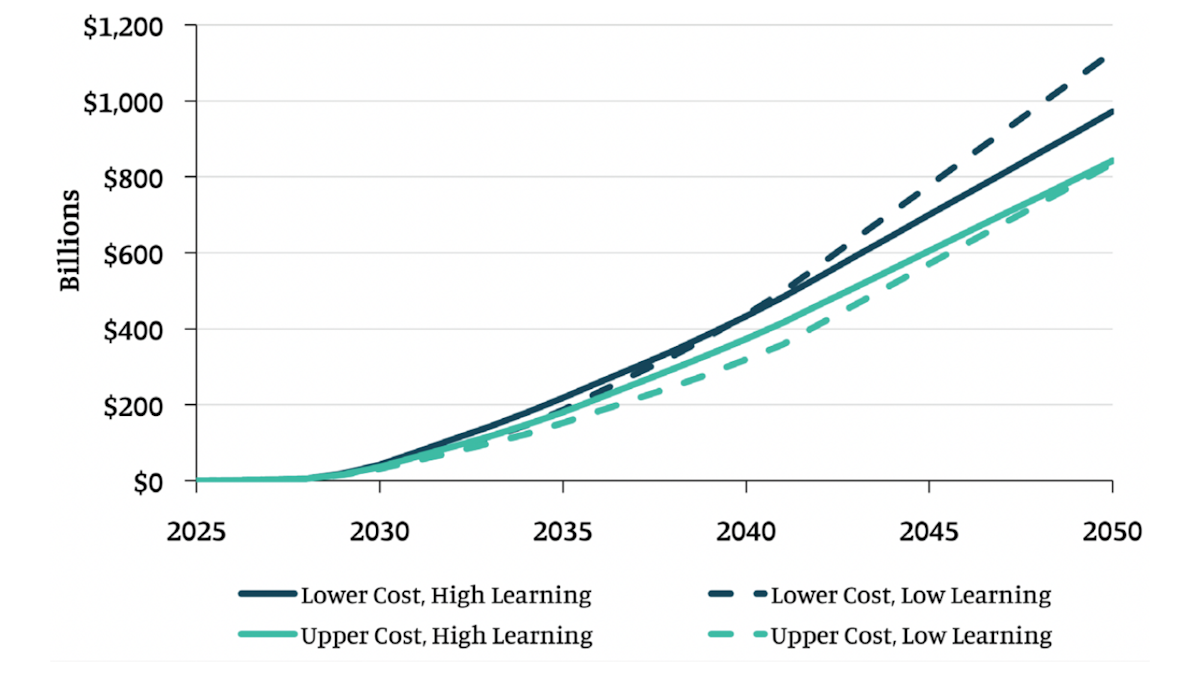
The following sections discuss various factors that will likely differentiate the financing and construction of advanced nuclear power plants from previous generations of traditional nuclear reactors. These considerations will ultimately determine the degree to which advanced nuclear designs are able to achieve cost improvements and more easily secure capital investment and financing.
3.2 Deployment and Capital Availability
To achieve cost reductions in key areas for advanced nuclear power, the industry will need to successfully develop a factory-scale construction program. Smaller reactors forgo some cost reductions achieved with the economies of scale provided by conventional large reactors. Instead of pursuing economies of scale, modular designs can achieve potentially significant cost reductions through the production of multiple reactors of a standardized design.
Achieving the scale of mass production necessary to unlock major cost improvements will not be possible if just a single demonstration project is completed and operated for years before subsequently scaling up deployment. An unsuccessful demonstration program or delays in the first wave of advanced nuclear demonstrations and subsequent early-stage projects will impede cost reduction from technological learning and restrict the potential for advanced nuclear to help meet US climate goals.
Given the importance of sufficient capital availability for early reactor builds, capital formation in both the public and private sectors is a necessary condition to achieve sustainable, cost-effective advanced nuclear energy deployment. However, under-investment in technology development and commercialization is typical, especially when large capital investments are required. The consequence is that new technology is often under-deployed. This is particularly true for innovations linked to the “public good”, such as addressing climate change, which is not fully valued in the market, making it difficult for private firms to capture sufficient benefits to make deployment financially attractive.
Cost reductions achieved through sufficient early investment and deployment of advanced nuclear power reduce the overall investment required (Figure 3-2). This results in a greater market opportunity by making the technology cost-effective enough to compete without the need to consider the unpriced public benefits.
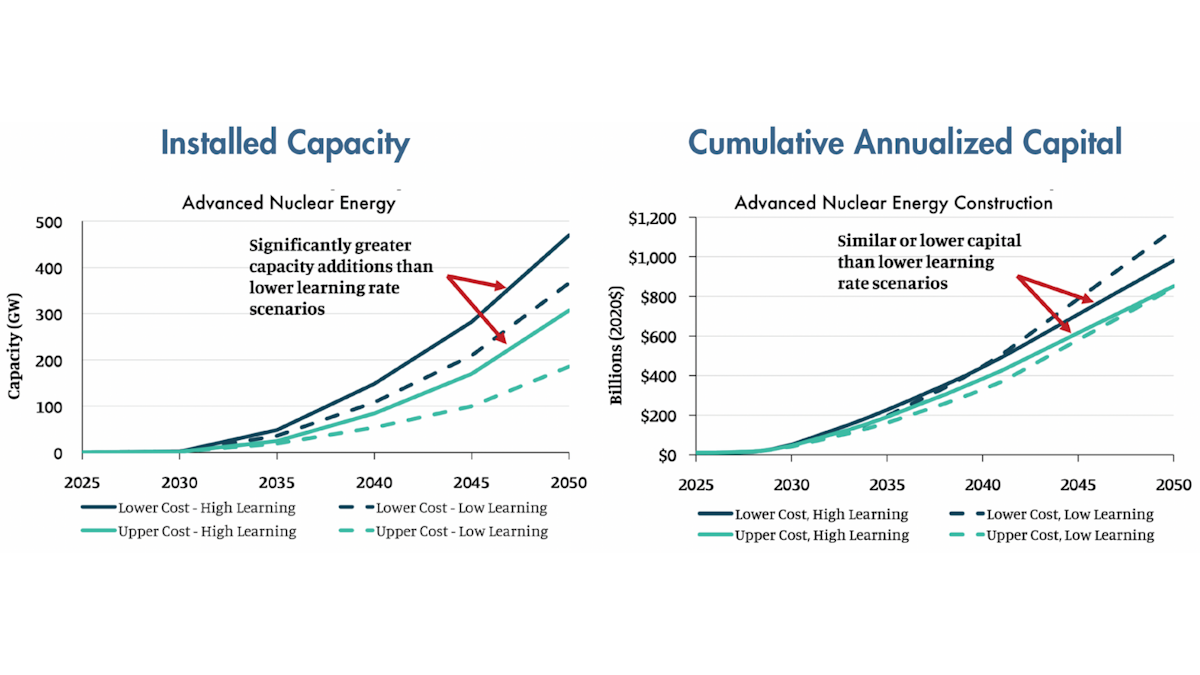
The clean firm power landscape is likely to be competitive starting in the near future. Other emerging clean firm power technologies such as NGCC+CCS, advanced geothermal, or run-of-river hydropower, may be able to fill the need for clean firm power on a decarbonizing grid, potentially competing with advanced nuclear reactors in some applications and contexts. Dispatchable utility-scale or distributed energy storage can also increase grid flexibility. However, each of these technologies has its own drawbacks, as does advanced nuclear. For example, this study assumes an optimistic CCS capability of 95 percent, which may be lower in practice. Enhanced geothermal increases the geographic options for siting geothermal power, but siting potential for enhanced geothermal systems remains somewhat restricted. Similar considerations apply to run-of-river hydropower, which is subject to even more limited siting constraints. High reliance on grid-scale storage to help match the daily generation profile to demand may substantially increase system costs. It is also assumed that there are no restrictions on HALEU fuel production, an assumption that will require active federal policy efforts to realize.
More broadly, increasing the available portfolio of clean firm power sources and encouraging competition among technologies will be important for decarbonizing not only the power sector but also the rest of the economy. Advanced nuclear, for instance, may play an important role in unlocking low-carbon solutions in other economic sectors, such as powering industrial processes and supplying residential and commercial heat (see Section 5).
3.3 Financing
Financing is expected to be easier for advanced reactors than for large conventional reactors because of their lower absolute cost, reduced construction timelines, and lower construction risks due to factory production. Although initial financing conditions (e.g., the cost of capital) are expected to be higher for the first advanced reactor unit, successful construction and operation of initial builds will likely improve conditions for financing subsequent projects. Such staged increases in capacity should reduce the financial risk associated with long-lead-time, capital-intensive projects.
Project structure and management could also help reduce capital costs per kWe for advanced nuclear. In traditional nuclear construction projects, the “stacking” or “pancaking” of contract contingencies and profit margins across a fragmented supply chain has escalated project costs over time. The increase in average capital costs per MWh for conventional light-water reactors in the last decade is mostly due to vendor/supplier agreements and risk management (increasing the cost by 70 percent), rising commodity prices (adding another 25 percent), and growing owners’ costs (about 17.5 percent). Together, these factors more than doubled the costs of traditional nuclear reactors between 2004 and 2011.
In contrast, advanced reactors are expected to rely on a more integrated supply chain and fewer subcontractors organized in fewer layers. Such improved organizational and management efficiencies have the potential to reduce the multiplication and stacking of contingencies.
3.4 Discount Rate
The discount rate is the opportunity cost of capital (as a percentage of the value of the capital). The opportunity cost of capital is the return on investments forgone elsewhere by committing capital to the investment under consideration. Higher discount rates negatively impact capital-intensive projects by increasing overall project cost and levelized cost of electricity (LCOE). Large nuclear energy projects have historically had higher discount rates compared to other technologies. Conventional nuclear power has been considered a higher investment risk than other conventional technology due, in large part, to past delays and cost overruns for nuclear projects. This additional risk is reflected in higher discount rates. Reductions in the discount rate are usually expected after multiple projects meet the expected cost and construction timeline, demonstrating their future potential for success.
Municipal or government-backed projects generally have a lower discount rate than investor-owned projects, reducing the overall project cost. Municipal projects usually have a higher credit rating than investor-owned entities, allowing them to secure lower interest rates. Further, they do not pay taxes or have to consider the return on equity.
Advanced reactor projects reduce investment risk compared to that of conventional reactors. Sequenced construction exposes less capital to loss due to cancellation at any given point in the project. Sequenced reactor projects are completed more quickly, enabling faster learning and reducing project risk. Multiple reactor developers are following an iterative innovation approach that reduces design and manufacturing risk before a full-scale design is available. Some developers plan to own and operate the reactors and sell the electricity directly on a contract.
3.5 Project Planning
Project design can significantly affect many investment parameters. Recent nuclear projects have experienced cost overruns during the construction phase, caused in part by project management inefficiencies. Nuclear energy projects are not alone, however; many large projects experience delays. , Moving to a standardized factory construction model with smaller units shifts project design to a smaller project paradigm, avoiding many of the challenges with larger projects.
Multiple Reactor Sites
Lower capital costs per reactor can be more readily financed. Sequenced construction also allows for a more distributed pattern of capital expenditure (Figure 3-3). This facilitates the capital formation and potentially enables access to capital at a lower discount rate for smaller utilities or customers with less capital on hand or limited ability to carry debt.
A more sequenced approach to construction can use construction resources more efficiently. The total site workforce can be reduced, moving from one reactor to the next as tasks are completed. Projects will also require a reduced pool of construction assets and equipment. If delays occur in the process of building one reactor unit, construction can begin or continue on the next module. This strategy presents numerous advantages over building a single large light-water project, in which a construction issue would force a larger group of workers to delay work until the issue is resolved.
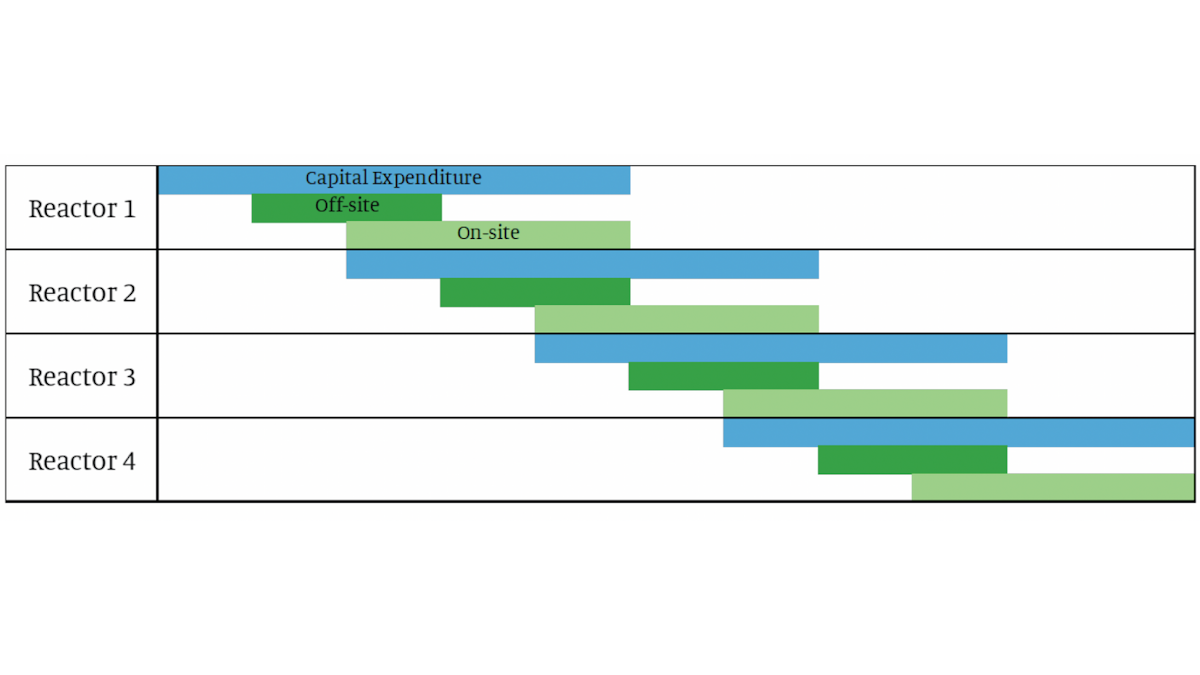
Multiple reactors at a site allow for additional cost savings from shared infrastructure, such as transmission, operations, facilities, and workforce. This advantage is well understood with existing large conventional power plants, and multi-reactor sites have remained more economical than single-unit sites.
Another important consideration for the advanced nuclear sector will be the appropriate scaling of upstream factory manufacturing capacity. Factories can operate more efficiently and cost-effectively when optimally designed to meet the needed level of demand from the industry at large. While a sequenced reactor construction approach can reduce project-level risk, parallel projects reduce the risk of lag or downtime (Figure 3-4).
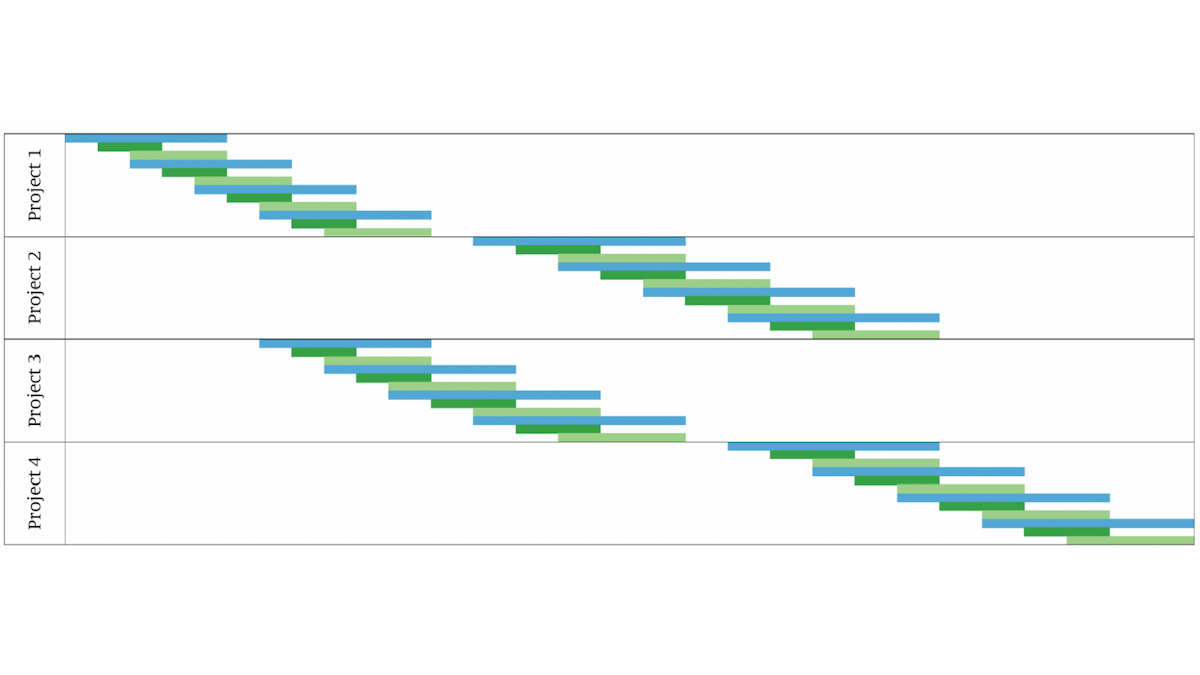
Orders for long-lead projects or limited manufacturing capacity are typically made far in advance, creating a queue or backlog. Backlogs are common in industries that specialize in large and complex products. The commercial aircraft industry provides several parallels to small modular reactor manufacture. Airliners are very complex, expensive, and safety-critical products that are constructed and assembled in a factory. Orders are commonly placed years in advance, and manufacturers carry a backlog of several thousand units.
A backlog of orders is useful to define the scope and initial priorities for manufacturing capacity, while also allowing for pre-planning of efficient manufacturing capacity expansion in the future. The backlog also provides a buffer to maintain steady production 一 even if order volume periodically drops. Sustainable backlogs are a positive indicator to investors. However, excessive backlogs signal the need to expand capacity to meet customer demand and maintain customer confidence that the order will be filled.
Early demand and orders for reactors will send a strong demand signal to prospective suppliers. A backlog of orders for reactors will provide justification for significant investment by companies that supply fuel and component manufacturing capabilities.
3.6 Economic Metrics
This study evaluates the costs of various energy technologies as a function of either deployment-driven capital cost improvements or projected capital cost curves, along with the costs of providing energy generation to meet grid dispatch requirements. The capital, fixed, and variable costs can be assessed together as the levelized cost required to produce a unit of electricity. Importantly, levelized costs decline both from capital cost reductions and capacity factor rates. Two levelized cost metrics are used to highlight how different clean firm power technologies provide value to the grid and how costs are expected to evolve over time.
Overnight costs
Reductions in OC from learning-by-doing can only be achieved if sufficient capital is mobilized and is done so in a timely fashion. Figure 3-5 shows the cumulative invested capital, translated from the modeled advanced nuclear deployment rates, needed to facilitate OC reductions and achieve a net-zero power sector by 2050. Importantly, the plotted capital formation considers capital deployment only for project builds. Supporting and ancillary advanced nuclear service industries, such as HALEU fuel production, require capital deployment well before power plant project capital mobilization. Capital formation needs to begin today, growing to around $30 to $40 billion of cumulative deployed capital by 2030 and $150 to $220 billion by 2035 in order to drive sufficient commercialization of advanced reactors to achieve necessary cost improvements. These investments are crucial for positioning the advanced nuclear sector to accelerate the rate of capital cost declines in the late 2030s.
Advanced Nuclear Capital Formation and Overnight Cost Across Scenario Ranges
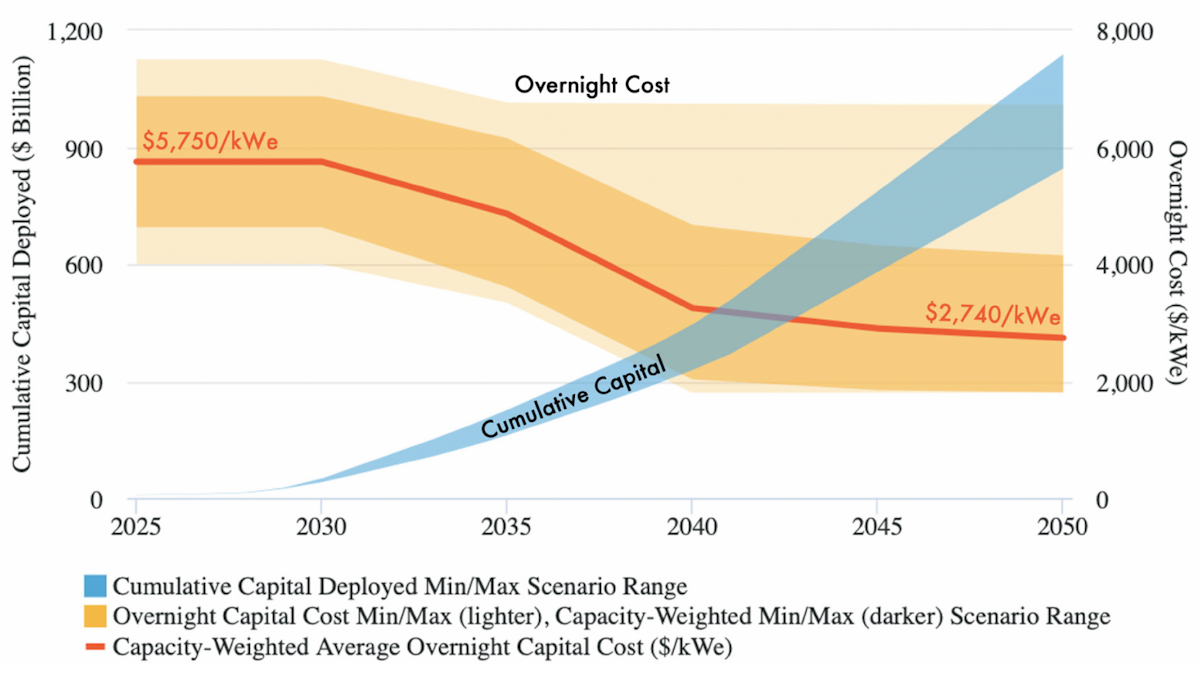
Levelized cost of electricity
OC learning manifests in substantial reductions in the LCOE for advanced nuclear power. Similar to Figure 3-5, Figure 3-6 plots improvements in the LCOE for advanced nuclear energy versus capital formation. The difference is that in Figure 3-6, LCOE (a generation-based metric) is plotted instead of OC (capacity-based), and as such, all weighted averages are weighted using generation instead of capacity.
Advanced Nuclear Capital Formation and Levelized Cost of Electricity Across Scenario Ranges
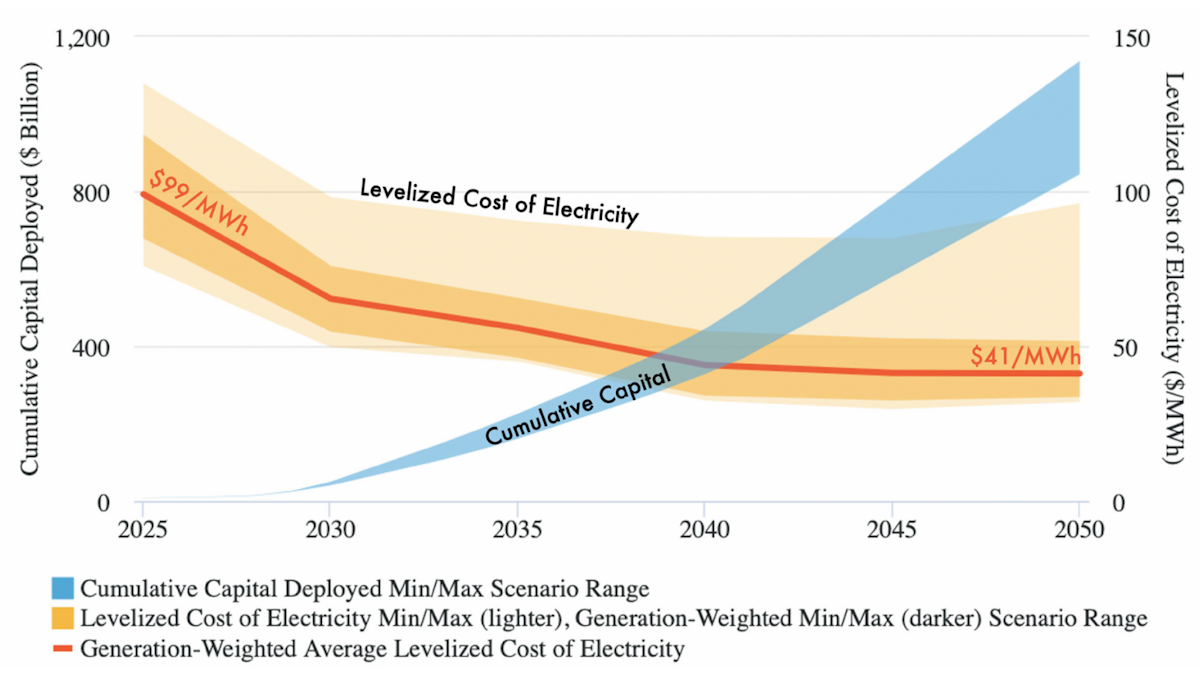
With advanced nuclear becoming a major component of the modeled US power grid by 2050, the cost of advanced nuclear power strongly influences the overall retail cost of electricity. The two Lower FOAK Cost model scenarios yield overall national average retail electricity prices of $59/MWh to $65/MWh in 2050, while the two scenarios with Upper FOAK Cost result in retail electricity rates of $76/MWh to $81/MWh.
While LCOE is an important metric for ascertaining the total cost of generation, it is by its nature significantly influenced by the average technology capacity factor. In particular, LCOE is composed of three cost components: capital costs ($/kWe), fixed annual costs ($/kWe•yr), and variable costs ($/MWh). Variable costs can be further broken down into fuel costs ($/MWh) and variable operations and maintenance costs ($/MWh). Equation 1-1 expresses the formulation for LCOE, segmented by levelized capital, fixed annual, and variable costs. Importantly, the average capacity factor is used to levelize capital and fixed costs.
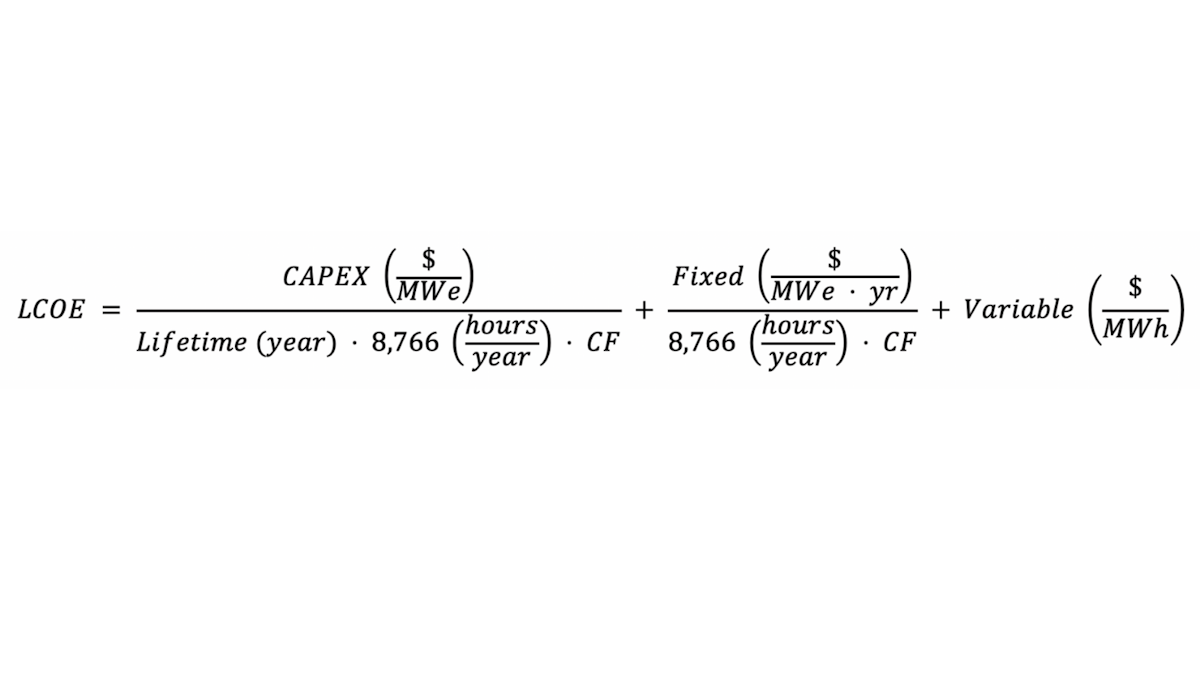
Evidence of the impact of capacity factor on LCOE is readily visible in Figure 3-6, where some inflections and even increases in the maximum LCOE appear despite monotonic, long-term reductions in capital and fuel costs. These variations are primarily driven by capacity factor changes as the grid decarbonizes and the utilization rate of different generating technologies shifts. We probe this phenomenon further in Figure 3-7.
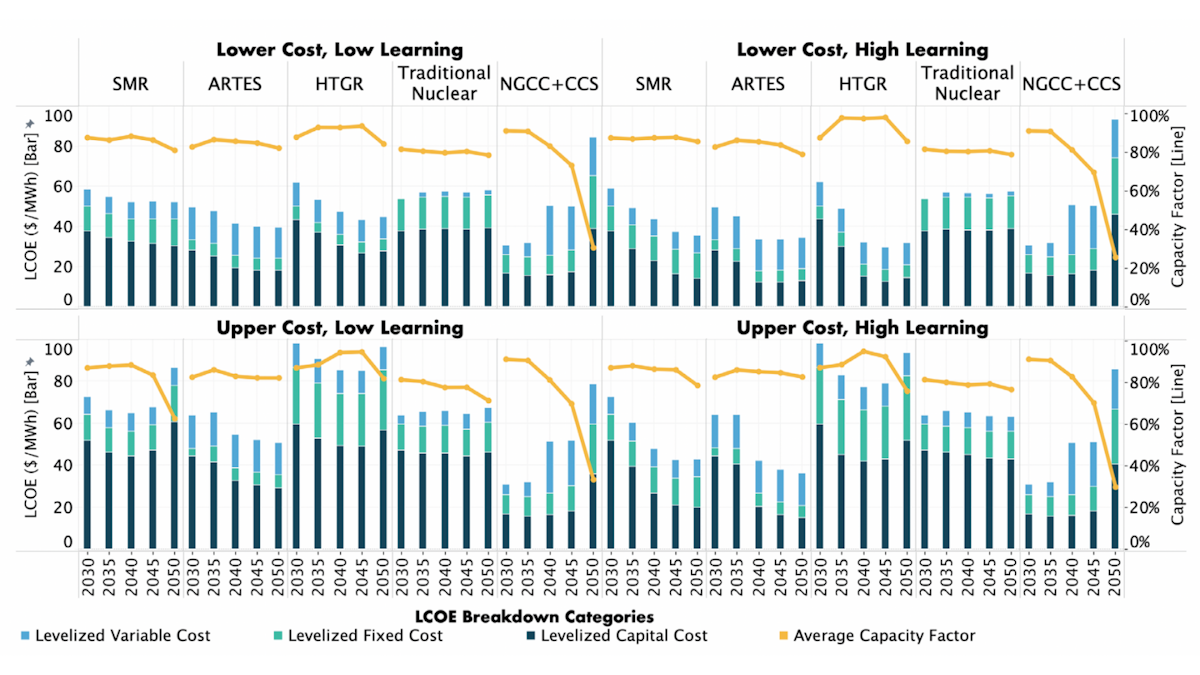
The key takeaways from Figure 3-7 are threefold. First, nuclear power LCOE is generally dominated by levelized capital costs, followed by levelized fixed and variable costs. And with the exception of ARTES reactors, levelized variable electricity costs represent a fairly minor contribution to LCOE for nuclear technologies. Second, advanced nuclear technologies typically achieve LCOE cost parity with NGCC+CCS by 2040. Third, the capacity factor substantially influences the LCOE. Despite OC reductions, levelized capital costs actually increase in certain situations as absolute OC learning begins to plateau and capacity factors dip. Importantly, the learning rate remains constant, but the successive OC reductions in absolute dollars decrease over time. The capacity factor of some clean firm technologies begins to decline in the late 2040s. This only marginally affects advanced nuclear technologies, but seriously impacts NGCC+CCS. Precipitous declines in average NGCC+CCS capacity factor between 2045 and 2050 due to economic pressures, result in sharp increases in levelized capital and fixed costs and a transition for these plants to a backup role for the grid. This is expected since, of the three constituent costs in the LCOE (Equation 1-1), the average capacity factor is only used to levelize capital and fixed annual costs, and these cost components are inversely proportional to generator utilization rates.
LCOE is only one instantiation of a levelized cost metric. For example, energy generation technologies can also be characterized using a capacity-based levelization method we call the levelized annual cost of capacity (LACC) [$/kWe•yr]. LACC reduces the influence of capacity factor on the levelized cost value because, unlike LCOE, its derivation includes capacity factor only to levelize variable costs.
In this way, a complete description of levelized costs for energy generation technologies should include both LCOE and LACC. And in particular, technologies with proportionally high variable costs are better characterized by LCOE because a low capacity factor does not significantly deflate overall LCOE. Similarly, energy technologies with proportionally high capital or fixed annual costs are better analyzed using LACC because a low capacity factor does not significantly diminish the contribution from capital and fixed annual costs. More details on the derivation of LACC and the application of LACC analysis to our modeled results are presented in Appendix D
4. Initial Target Markets and Customers
Over the past decade or more, researchers, policymakers, and industry have proposed a large number of potential contexts in which advanced nuclear reactors might be commercially deployed. , Such suggestions have often been speculative and warrant more careful evaluation to distinguish between viable opportunities for early deployment in the nearer term and applications that would face more substantial obstacles in terms of cost-competitiveness or other barriers.
In this section, a number of settings and contexts are theorized to offer strong market potential for future advanced microreactor or small reactor deployments and assess the advantages offered by nuclear energy production relative to competing options and to potential obstacles to deployment. Proposed initial target markets and customers are classified into two broad categories: early stage and intermediate stage. Early stage markets and customers are approximately those potentially best suited for FOAK to NOAK advanced nuclear projects during the very opening phase of technology commercialization. In contrast, intermediate stage markets and customers face additional cost, technical, regulatory, or readiness concerns that may make them better suited for a subsequent phase of deployment.
4.1 Early Stage
Existing Nuclear Sites
Suitability: Conventional and Small Reactors
Sites of existing or retiring nuclear power generation may provide favorable opportunities for initial advanced nuclear projects. A strong precedent in the United States exists for the construction of new nuclear units of a different design at existing nuclear power plants. Examples include the Peach Bottom Atomic Power Station in southeastern Pennsylvania, the Dresden Generating Station in northeastern Illinois, and the Alvin W. Vogtle Electric Generating Plant in northeastern Georgia. Existing nuclear power plants have already been proposed as candidate sites for the Advanced Reactor Demonstration Program (ARDP). Existing and retiring nuclear power plants make appealing locations for advanced reactor projects as they already meet regulatory criteria ranging from seismic stability to the siting of emergency planning zones. Furthermore, local stakeholder considerations may be facilitated by community comfort and familiarity with nuclear technology.
Replacement of Coal, Gas, and Oil-Fired Power Units
Suitability: Small Reactors
To-be-decommissioned and recently decommissioned coal-fired power plant sites and other fossil fuel generating stations represent desirable target locations for advanced reactors for several reasons. The repowering of coal and other fossil sites can help save early novel reactor deployment costs by allowing for some reuse of equipment and infrastructure. One recent academic study quantified potential savings of up to 15 percent on initial OC for an SMR sited at a decommissioned coal power plant, thanks to the ability to repurpose existing assets like cooling systems, switchyards, and grid connections. Existing road and rail connections may facilitate construction, while coal plant sites may also prove favorable for meeting regulatory siting guidelines. The Idaho National Laboratory has recently released an extensive report on the technical and economic considerations relevant to the repowering of coal-fired power plants with advanced reactors.
Repowering existing coal sites can generate continued local economic activity and employment, thereby appealing to local communities and stakeholders. The potential for retention of fossil plant workers, however, depends critically upon the time frames over which fossil plants are retired relative to the start of construction and operation of new onsite nuclear capacity. The potential scale, timing, and implications of repowering existing fossil-fired power stations nationwide with advanced nuclear reactors is discussed in Section 7.6.
Military Bases and Portable Military Applications
Suitability: Microreactors and Small Reactors
The US military could emerge as an early customer for micro- and small reactors. Two separate Department of Defense projects are already in progress. Operational considerations such as performance, reliability, security of fuel supply, and modularity can incentivize the pursuit of projects at a higher cost point than might be viable for many civil or private sector applications. Energy-dense, mobile, and reliable sources of power could also support the Department of Defense’s interest in the development and future deployment of laser and microwave weaponry with power levels ranging from 150 kWe to 1 MWe. However, any military requirements for special customization to meet specifications could complicate manufacturing and supply chain considerations.
Data Centers
Suitability: All Reactors
Electricity represents a major component of data center operating costs, and data centers also place a high value upon reliability and predictability of power supply. Some exploratory efforts are already underway to investigate connections for new data infrastructure with existing nuclear power plants. But as is the case for heavy industrial customers, data center operators are likely to prioritize electricity costs and risk reduction and may not be likely to emerge as early adopters of new advanced nuclear reactors. Given that the primary competitor in this end-use case is the price of conventional electricity, onsite nuclear could struggle to be considered for such applications.
At the same time, some large US-based technology companies such as Google and Microsoft have signaled strong interest in procuring 24/7 carbon-free electricity to power their business operations and activities as part of long-term corporate climate commitments, even if such clean electricity comes at a premium cost. As such, these and similar voluntary commitments by tech companies could potentially drive greater adoption of advanced nuclear technologies for data center applications than would otherwise occur due to cost factors alone. A preliminary agreement by advanced reactor developer Oklo to partner with a cryptocurrency firm suggests the possibility for earlier adoption in some applications.
Remote Communities
Suitability: Microreactors and Small Reactors
Many remote and island communities rely on fossil energy generation and imported fuels, facing both high energy costs and vulnerability to supply shortages. Most remote communities have power requirements of 5 MWe or less and are thus promising candidates for microreactor-sized projects, while small reactors may prove well suited for replacing fossil generation in larger towns or small cities in remote regions. Microreactors may be able to achieve cost-competitiveness with diesel power generation in remote communities even from the very outset of early deployment. , Island communities often exhibit a similar reliance on fossil generation and fuel imports, but enjoy access to maritime shipping and thus confront lower costs and risks of supply disruption. At the same time, land scarcity and the value of potential desalination via the co-generation of heat strengthen the value proposition for nuclear power over other generation technologies.
Direct Contracts
Suitability: Microreactors and Small Reactors
Direct sourced contracts and power purchase agreements are used to purchase electricity from a single supplier. These contracts are common in industrial and commercial applications where the buyer desires to reduce market cost volatility and ensure supply. Increasingly, companies are also using these contracts to directly purchase electricity specifically to meet internal goals of consuming only clean energy. Early efforts have primarily focused on solar and wind renewable energy credits. However, these sources do not generate electricity that is directly matched to consumption. Clean firm energy sources, like nuclear energy or geothermal, will be required to meet hour-matched consumption.
4.2 Intermediate stage
Off-Grid Extractive Industrial Sites
Suitability: Microreactors and Small Reactors
Remote industrial sites such as mines, logging operations, oil and gas operations, and water and wastewater facilities often face similar high energy costs and supply challenges as those of remote communities. Such customers are gaining focus as a potential area for early adoption of new nuclear technologies. For instance, the mining sector has expressed interest in the use of small reactors to decarbonize diesel generators currently used to power on-site mining equipment. Remote industrial applications would also particularly favor mobile or transportable reactor designs that could be relocated to new sites. However, industrial customers are likely to be both highly cost-conscious and risk-sensitive. Many of these industries already face considerable operational uncertainties and tight profit margins. As such, extractive sectors are likely to wait to observe the results of initial deployment efforts elsewhere before committing to adoption.
Industrial Heat, Power, and Co-Generation
Suitability: All Reactors
The heavy industrial and manufacturing sectors remain major consumers of electricity as well as fossil energy for industrial heat. For many sectors including but not limited to steel, aluminum, computer chip manufacturing, shipbuilding, and petrochemicals, energy costs represent a sizable fraction of overall costs. Particularly as some industrial commodities like steel and aluminum face the prospect of carbon border taxes that may impose trade tariffs on carbon-intensive products, major industries are increasingly prioritizing innovative ways to reduce carbon intensity. Small reactors are often proposed as an appealing solution to meet clean energy requirements for many industrial activities. Co-production of heat can also serve a versatile array of industrial applications including hydrogen and ammonia production. Yet despite the potential value of nuclear energy for industrial customers, cost considerations and uncertainty regarding unproven technology are likely to dissuade industrial customers from early adoption. Pilot programs with public sector support, such as a recent $10 million US Department of Energy award to Xcel Energy to explore nuclear hydrogen production, could accelerate the pace of adoption.
Transportable Designs
Suitability: Microreactors and Small Reactors.
In addition to the military and off-site industrial applications mentioned above, some have suggested that mobile advanced reactor designs could also provide long-term power to small communities or could be designed for rapid-response deployment during emergencies or natural disasters. However, the commercialization of mobile, transportable reactors will critically depend on the development of new regulations to govern this category of nuclear technology—an unpredictable process that may take many years. This factor complicates any potential for the near-term commercialization of transportable reactors intended for highly flexible siting and operation.
Moving forward, the use of nuclear energy for marine-based propulsion could expand given the few available options to decarbonize propulsion for large ships. However, the unique regulatory uncertainties associated with marine-based small reactors, in conjunction with the high costs and long lead times of the domestic shipbuilding sector, make marine-based nuclear applications a particularly poor candidate for targeted deployment in the short term.
5. Non-Electric Revenue
More efficient use of low-carbon energy beyond the power sector will optimize future energy systems design to meet ambitious national decarbonization goals. Developers and researchers are exploring numerous additional applications for advanced nuclear energy and potential co-products including hydrogen production, desalination, heating, and others. These additional uses may also provide multiple additional revenue streams that could enhance the profitability of nuclear power and expand the range of markets in which nuclear technology can be applied.
Useful co-products of nuclear energy can also provide valuable social and economic benefits to nearby communities. Key energy system and community benefits of advanced nuclear are discussed in the subsequent major report section.
Expansive, versatile non-electric applications help position nuclear energy as an extremely valuable multi-sector solution for not only national but global clean energy needs. As worldwide energy demand continues to rise, with emerging economies becoming wealthier and improving population-wide living standards, energy access and energy consumption will likewise increase. Particularly given the value of non-electric co-generation, advanced nuclear technology possesses considerable international market potential and presents a rich opportunity for American technology and innovation to regain a leadership role in the global energy technology marketplace.
5.1 Industrial process heating
Nuclear energy not only provides clean electricity but also produces heat as a reliable, non-fossil option for industrial process heating. Some advanced reactor designs feature high-temperature characteristics particularly attractive for industrial applications. For example, a 2011 Idaho National Laboratory (INL) analysis demonstrated that a high-temperature reactor (HTR) multi-module plant could support high-temperature processes at prices similar to those of natural gas in the price range of $6-$9/GJ. Different reactor designs offer diverse temperature ranges for a variety of industrial processes. For instance, LWRs are well-suited for lower temperature applications up to 300℃, while Gas-cooled Fast Reactors (GFRs), molten salt reactors, and HTGRs achieve temperatures over 500℃ for high-heat applications.
Industrial processes that use heat include methane reforming, thermal water splitting for hydrogen production, steel and cement manufacturing, paper production, and more (Figure 5-1).
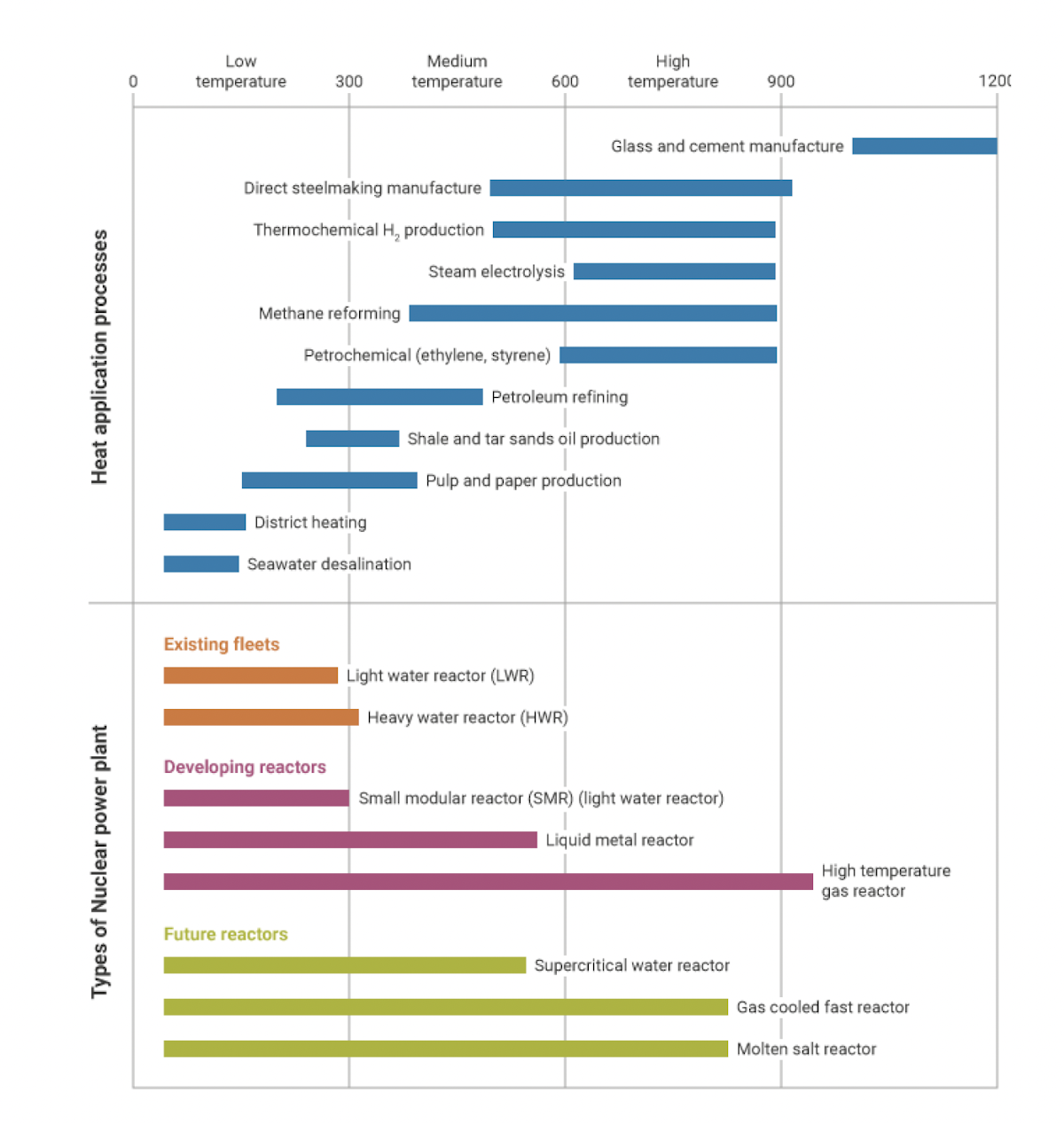
HTGRs have the most market potential for supplying industrial heat applications. One study estimated that within the current market, the potential market for nuclear process heat sums up to a total heat load of 131,231 megawatts-thermal (MWth) (equivalent to approximately 1,035 TWh) per year based on reactor sizes of 150 MWth and 300 MWth with heat as the primary application. For context, this would be around 17% of the total installed advanced nuclear capacity (equivalent of 763,000 MWth) in the year 2050 for our Upper Cost, High Learning scenario.
5.2 District heating
Zero-carbon heating sources will play an increasingly prominent role in the future for meeting greenhouse gas reduction targets. Nuclear energy is a promising option for supplying district heat for domestic applications — a productive use of clean heat that would otherwise go wasted.
District heat from nuclear power is used in many locations throughout Europe and Russia, with over 750 combined operating years of experience. In the summer of 2021, for instance, the China National Nuclear Corporation (CNNC) launched a district heating demonstration project for the Qinshan nuclear power plant. This initiative will supply heat to a total of 464,000 square meters of residences in Haiyan County, Zhejiang province. A 2018 European study evaluated 15 district heating system models using nuclear for combined heat and power plants with an assumed 25% heat connection rate. Researchers found 7 systems were cost-effective and could decrease annual fossil emissions up to 10 Mt CO2.
Due to their reduced size and improved safety characteristics, advanced nuclear reactors may be more flexibly sited closer to small population centers in the future, increasing the potential for advanced nuclear power to serve urban heating needs in towns or small communities like college campuses.
District heating systems are generally less common in the United States than in Europe, Russia, or East Asia. Nuclear power is an untapped resource for its domestic expansion. There are currently only 660 district energy systems operating in the US, many of which are operated by communities, universities, hospitals, and airports such as Cornell University and the New Orleans Regional Medical Center District Energy System. , , Millions have been allocated to expand district energy systems supplied by renewable energies, such as the South Loop District’s proposed district heating systems in Bloomington, MN. , Advanced nuclear reactors could supply the electrical grid while providing carbon-free heat to such systems.
5.3 Desalinization
Potable water sources are limited in certain regions of the United States, including coastal areas. Desalination of seawater involves pumping seawater through filters and other treatment processes that consume considerable energy, which makes it costly. Nuclear-powered equipment could reduce desalination costs and carbon emissions, either in conjunction with pre-existing infrastructure or as a complementary feature of new power plants.
The average cost of desalination has fallen within the past two decades and is expected to continue falling for the next 20 years, making desalination more competitive for meeting public water supply needs. The local urgency ignited by increased domestic migration to water-stressed urban areas such as the United States Southwest is giving some regional governments and municipalities acute reasons to explore alternative solutions. San Diego, for instance, receives half of its water from the Colorado River, which has been decreasing in volume in tandem with decreasing snowfall from the Rocky Mountains. New desalination capacity may solve challenges elsewhere, including globally, helping meet long-term water demands and presenting another opportunity for efficient, zero-carbon new nuclear energy technologies.
Desalination can be accomplished at lower temperatures than most of the other co-generation processes discussed in this report. Suitable nuclear plants for desalination systems include even low-temperature LWRs, heavy water reactors, and SMRs in temperature ranges of 280-325°C. Small and medium-sized nuclear reactors may be ideal for optimizing desalination capacity. , Cost estimates for desalination technologies based on country case studies are shown in (Table 5-1) below, converted into 2022 dollars.
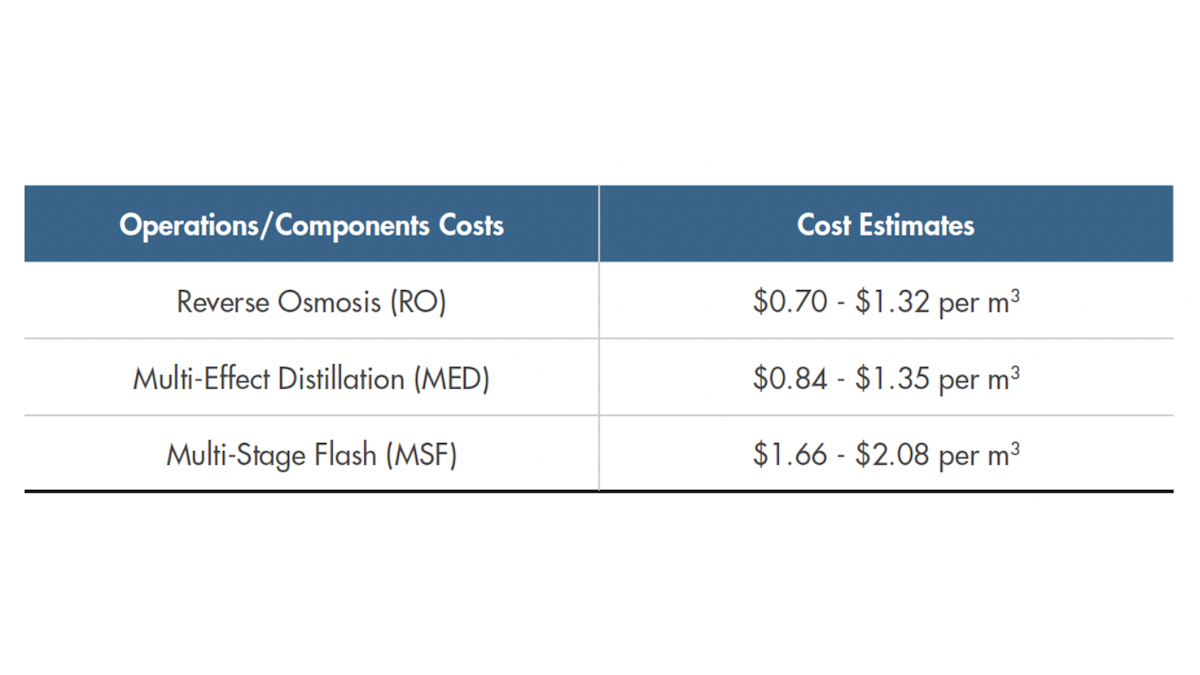
The presence of an existing nuclear power plant may significantly improve the economics of future desalination investments. For instance, a 2021 study by Stanford and MIT showed that using the Diablo Canyon nuclear power plant in California to power desalination systems could provide fresh water in quantities equal to or more than a network of waterways throughout California (Delta Conveyance Project) while requiring substantially lower capital costs. , Such considerations emphasize the value of broad, multipurpose planning around advanced nuclear deployment in the coming decades.
5.4 Hydrogen
An added benefit of nuclear energy is its potential to power large-scale hydrogen production. This is generated through electrolysis, particularly during periods of lower electricity demand. Future hydrogen production from nuclear sources could also adopt emerging new approaches, such as the decomposition of water using direct heat from the reactor. Hydrogen fuels some large-scale, power- and heat-intensive industrial processes (e.g., oil refining and ammonia production), presenting opportunities for advanced nuclear power to cleanly and reliably power hard-to-decarbonize sectors of heavy industry.
Numerous demonstration projects are underway throughout the United States to explore hydrogen production from nuclear power and nuclear process heat. These efforts include but are not limited to:
The Department of Energy (DOE) in October 2021 allocated 20 million in funding to produce hydrogen at Palo Verde Nuclear Generating Station in Phoenix, Arizona.
- The Hydeal Los Angeles project plans to convert natural gas plants to hydrogen-powered plants to achieve LA’a net-zero goals by 2035.
- In 2020, The DOE awarded Xcel Energy $10 million to investigate hydrogen production from steam electrolysis in collaboration with INL.
- Exelon aims to produce hydrogen at the Nine Mile Point Nuclear Station using a Proton Exchange Membrane electrolyzer.
- Energy Harbor is producing hydrogen using Low Temperature Electrolysis and Polymer Electrolyte Membrane.
Nuclear-powered hydrogen production represents an additional revenue stream for new and advanced nuclear technologies. Indeed, some research efforts are focusing on nuclear plant designs that incorporate electrolytic hydrogen production using nuclear process steam into a multigenerational system in conjunction with co-located solar PV installations that provide cheap electricity.
Hydrogen production from clean nuclear power could offer benefits that other clean energy sources do not. For example, a Stanford-MIT study found that Diablo Canyon could produce 110 kg of hydrogen annually at a per-unit cost within a range of $2.01 - $2.46/kg H2, which is half the cost of production from solar and wind today at a fraction of the land footprint. A 2020 report by Lucid Catalyst projected that next-generation SMRs could potentially achieve hydrogen co-production for as little as $0.90/kg by 2030.
5.5 Synthetic fuels
Net-zero synthetic fuels are another asset that advanced nuclear reactors could potentially produce. The Electric Power Research Institute (EPRI) explored the feasibility of advanced nuclear designs powering floating-production, storage, and offloading facilities (FPSO). While no nuclear-powered FPSOs are in operation today, marine vessels have long harnessed nuclear propulsion, providing a wealth of operating experience for nuclear-generated power. Meanwhile, maritime applications for conventional FPSOs are similarly well-established.
To advance a low-carbon economy, hydrocarbon fuel could be produced from limestone using electrolysis, without the emission of carbon dioxide. EPRI’s cost estimates suggested that nuclear-powered, marine-based FPSOs could shave construction costs relative to land-based facilities, with OC of $2200/kWe for a 600 MWe plant and $1600/kWe for a 1200 MWe plant. This estimate assumed a world-class South Korean shipyard and the participation of major manufacturers and suppliers with power sector infrastructure familiarity.
Given the tremendous relative decline in the capacity, economic competitiveness, and technological level of the United States shipbuilding sector over recent decades, domestic construction of nuclear-powered FPSOs would likely be unfeasible and involve prohibitive financial risk. The restrictions outlined in the Jones Act would also complicate the operation of foreign-built FPSOs off US shores. As such, marine-based synthetic fuels production likely only represents an opportunity for US advanced nuclear vendors insofar as they can compete to provide marine nuclear propulsion units for foreign-built FPSOs intended for foreign markets.
On shore, companies are already exploring land-based co-production of synthetic fuels with nuclear power, such as a Royal Dutch Shell initiative aiming to produce synthetic jet fuels from existing nuclear plants. The company has targeted production of up to 50,000 tons of synthetic fuel annually for Scandinavian Airlines once the production facility is commissioned between 2026 and 2027.
Comparison to Other Net-Zero Studies
6. Comparison with Nuclear Deployment Assessments in Other Recent Modeling Studies
Recently published decarbonization pathways studies have estimated future nuclear energy deployment under various cost and policy assumptions. This section summarizes nuclear sector findings from three major decarbonization pathways studies: Princeton University’s “Net-Zero America” (2021), Vibrant Clean Energy’s “Zero by Fifty” (2022), and Williams et al.’s “Carbon-Neutral Pathways for the United States” (2021). Each of these studies examines multiple scenarios that vary input assumptions and produce a range of nuclear deployment outcomes.
Unlike most decarbonization pathways studies, which do not fully recognize the costs and characteristics of advanced nuclear reactors , this modeling analysis uses cost assumptions that are tailored specifically to advanced nuclear technologies and grounded in updated cost estimates and operational characteristics published in the literature and obtained from reactor developers. Furthermore, endogenous learning rates are incorporated to reflect the dynamic relationship between costs and the deployment of novel nuclear technologies.
6.1 Energy System Results and Nuclear Technology Assumptions
Contrasting the Princeton Net-Zero America (NZA), Vibrant Clean Energy (VCE) Zero by Fifty, and Williams et al. study findings with one another and with this study reveals the importance of selected assumptions around advanced nuclear costs and operational characteristics. Notably, the inclusion of advanced nuclear designs in the capacity expansion model appears to considerably alter the composition of the energy system landscape.
By 2050, the US power grid operates a large installed capacity of advanced and conventional nuclear reactors across the four model scenarios in this study (247 to 489 GWe) and in the VCE Zero by Fifty models that permit advanced nuclear construction (293 to 473 GWe). None of the scenarios in these studies constrained the future deployment of renewables or selected for nuclear. This suggests that simply including advanced nuclear reactors as an option for the clean energy buildout can result in substantial advanced nuclear deployment as part of a least-cost pathway to a decarbonized power sector.
In contrast, the Princeton NZA and Williams et al., 2021 studies only considered traditional nuclear power plants as an option for new generating capacity, and their models thus build little or no new nuclear capacity except in scenarios where renewable capacity is limited by land or deployment rate constraints. New nuclear construction is economical only in these renewables-constrained scenarios, yielding an installed nationwide nuclear capacity of 310 GWe in the renewables-constrained Princeton NZA case and 150 GWe in the analogous Williams et al. case. At the same time, these two studies found that scenarios excluding nuclear and gas generation with CCS lead to higher total system costs and nationwide land use (see Sections 6.2-6.3 and Appendix D), highlighting how traditional nuclear energy plays a valuable role for energy system optimization even at present-day costs.
Indeed, the Princeton NZA and Williams et al., studies assume traditional nuclear capital costs on the order of $6,500/kWe, which are on par with initial capital costs for advanced reactors in the Upper Cost model scenarios, but well above initial costs in the Lower Cost scenarios. More importantly, advanced reactors possess different operational characteristics, such as improved ability to ramp up and down in response to grid-balancing needs, higher thermal efficiencies, and more flexible geographic siting (see Appendix A). One category of advanced reactors included in this study incorporates thermal energy storage capabilities, providing valuable additional generation flexibility and improving real-time market competitiveness (See Section 2.1 and Figure 2-4).
Overall, our results suggest that the cost improvements and favorable operational characteristics of advanced nuclear plants play an important role in encouraging new nuclear projects in a least-cost pathway to zero-emissions power.
6.2 Total System Costs
Major differences in study boundaries, scenario construction, modeling approaches, and methodology complicate some direct comparisons among these studies, particularly for certain factors like system costs. Nevertheless, an analysis of electricity sector and energy/industrial sector costs for these modeling studies produces some useful conclusions.
First, within the VCE Zero by Fifty, Princeton NZA, and Williams et al. analyses, those scenarios that allow the system to retain existing traditional nuclear generation, permit fossil fuel generation with carbon capture, and allow new nuclear power plant construction result in lower system costs overall and exhibit more favorable trends in system costs over time (Figure 6-1 and Figure 6-2). The inclusion of advanced nuclear technologies in some VCE Zero by Fifty scenarios (and in this study) effectively makes a new clean energy source available that can be flexibly sited while providing high system value. In contrast, the scenarios in the VCE, Princeton, and Williams et al. studies that prohibit new nuclear power plants or gas generation with CCS are the most expensive decarbonization pathways. This emphasizes the importance of pursuing a diverse and robust portfolio of clean firm generation options for building a decarbonized, cost-efficient power sector.
Second, comparing this report’s modeling results with those from the VCE Zero by Fifty study, both of which employed the same electricity sector model, shows that this study achieves similar system costs per unit of electricity generated and a faster rate of power system cost improvements over time (Figure 6-1). This occurs despite the model runs in this analysis employing higher overall technology and fuel cost assumptions than the VCE Zero by Fifty study, hence their higher calculated system costs of power production even from the start of the modeled period. Even so, the two Lower Cost scenarios have similar generation-normalized power system costs as the VCE study by 2050. Relative to power sector costs in 2020, the four model runs in this study exhibit cost improvements over time that are similar to (Upper Cost) or greater than (Lower Cost) those achieved by the published VCE Zero by Fifty model runs, suggesting that these four scenarios are cost-efficient.
Annual Electricity System Cost and Cost Ratio Over Time, Normalized to Generation
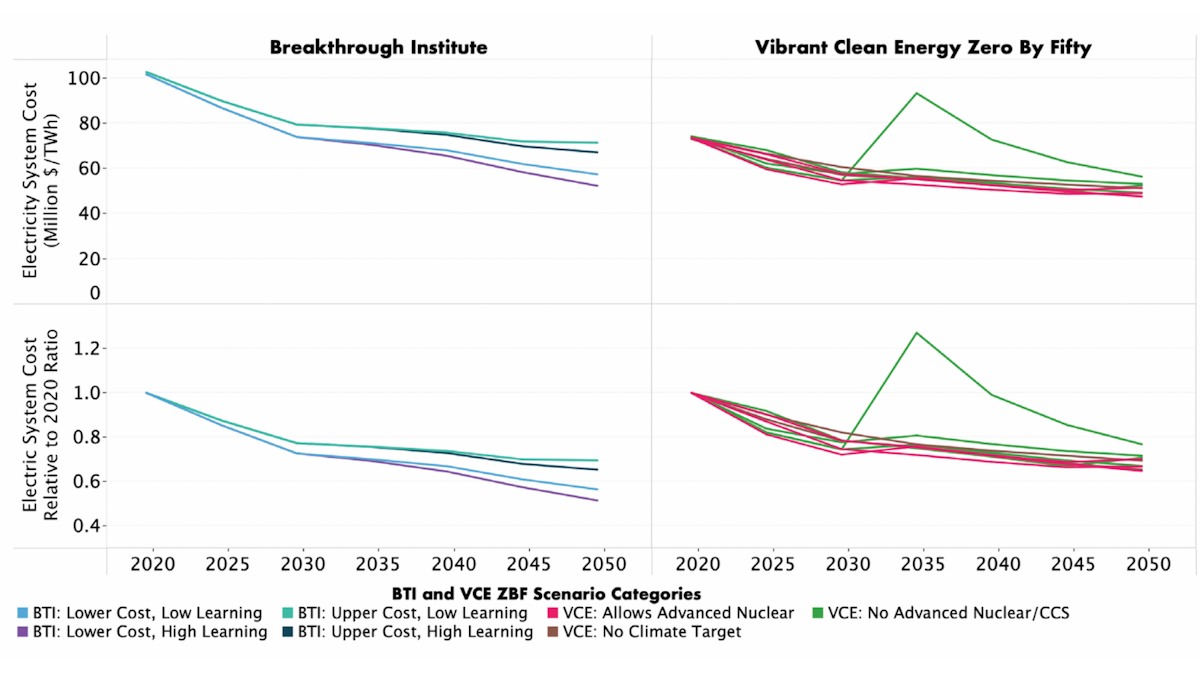
Total Annual Electricity System Cost and Cost Ratio Over Time
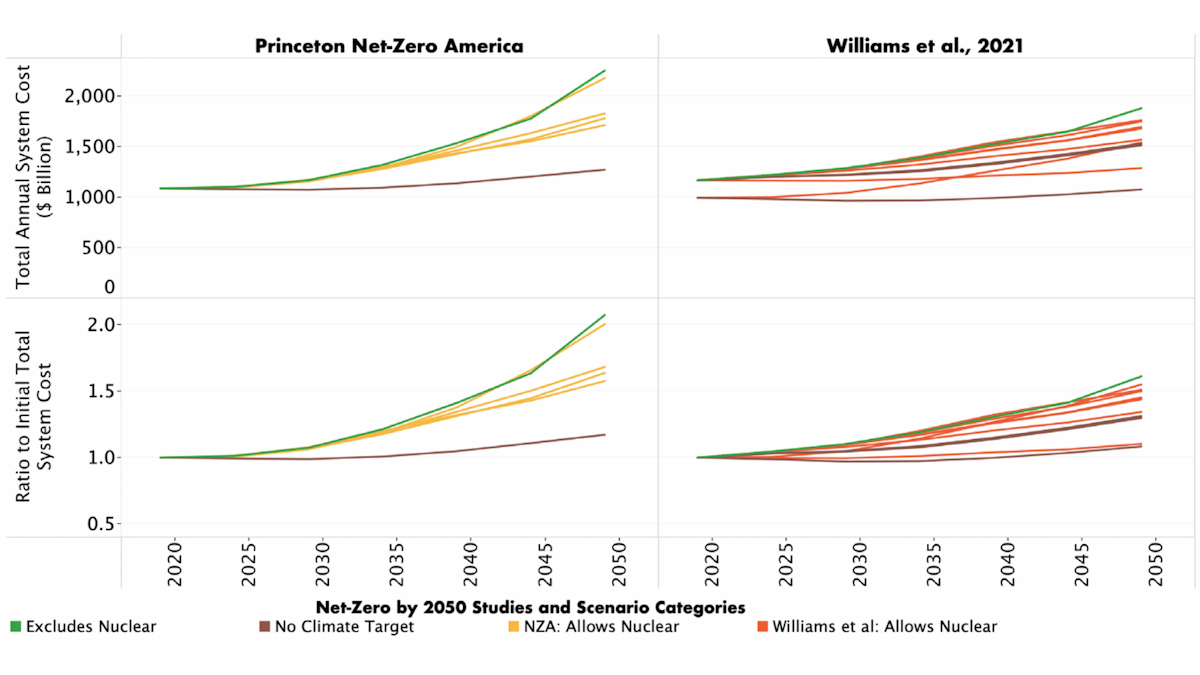
Note that the Princeton NZA and Williams et al. studies consider decarbonization and associated costs across the energy and industrial system as a whole, rather than just those costs associated with the power sector. This wider total system scope results in overall escalation of system costs over time (Figure 6-2), due to expenditures related to the steel sector, gas and CO2 pipeline infrastructure, end-user efficiency improvements, and other costs outside the power sector. The costs and cost trends in Figure 6-2 are also total costs, not normalized to electricity generation.
6.3 Land Use
Future clean electricity grid infrastructure will occupy a far larger total land area than the energy system today. While the direct footprint of wind turbines and associated roads and structures is less than 1 percent of the total area of a wind farm, multipurpose use of unoccupied land is nevertheless restricted due to permitting requirements that mandate minimum setbacks around turbines in the event of structural failure. In contrast, for utility-scale solar installations, the land directly occupied by solar modules represents a much higher fraction of the total solar farm area. A higher proportion of point-source clean energy generation like nuclear power, geothermal power, and gas with carbon capture can reduce the future land footprint required for solar and wind installations. Should future land availability constraints strongly limit the extent of wind and solar installations, such an economic and policy environment will likely further increase the value of advanced nuclear, geothermal energy, and fossil generation with CCS.
Modeled future utility-scale wind and solar land-use estimates allow for fairer direct comparisons among these four studies, as the underlying assumptions are highly similar. Across the four model scenarios in this study, a total cumulative land area of 182,000 - 277,000 square kilometers (18.2 - 27.7 million hectares) nationwide is occupied by wind and solar farms. This is equivalent to slightly more than the total area of Colorado at the upper end, and a little larger than Missouri at the lower end. This corresponds to less than 35 percent of the modeled total land use for wind and solar power estimated by Princeton NZA (51 to 107 million hectares in scenarios where renewables deployment was not constrained) and also falls below the range modeled by Williams et al., 2021 (29 - 47.7 million hectares in non-constrained scenarios).
The Princeton NZA and Williams et al., 2021 scenarios that prohibited nuclear and carbon capture technologies produced the largest future land use estimates, due to the far more extensive wind and solar buildouts needed to meet decarbonization requirements. The VCE Zero by Fifty land-use figures for wind and solar installations have not yet been publicly released.
6.4 Limits to Intercomparison
Aside from the choice of bounding nuclear cost assumptions and inclusion of realistic operational parameters, there are major differences between this modeling analysis and the three previously-published studies—even relative to the VCE Zero by Fifty study, which also uses the WIS:dom-P model. These considerations complicate attempts to directly compare and contrast this report’s findings against the prior work summarized above.
Unlike the Princeton NZA and Williams et al., 2021 studies, which use the EnergyPATHWAYS (EP) and Regional Investment and Operations (RIO) models, this study employs an entirely different capacity expansion model with a different spatial resolution, logic for power plant siting, weather-dependent solar and wind resource modeling, and many other factors. Boundaries for certain cost categories may not necessarily match, potentially resulting in varying definitions of outputs like system costs or clean energy jobs. A careful decomposition of each energy system cost component would be required to more accurately compare total system costs for these analyses. This is even before considering scenario-based differences such as input cost assumptions, power plant operational parameters, national electricity and energy demand, and different decarbonization targets.
This study also utilizes a number of assumptions and settings that differ from those used to produce the VCE Zero by Fifty results published to date, despite using the same underlying model. First, the VCE Zero by Fifty study targets economy-wide net-zero greenhouse gas emissions by 2050, whereas our study achieves net-zero power sector CO2 emissions by 2050. In addition, this study was also initialized in 2020 whereas the Zero by Fifty study was initialized in 2018, meaning that this study’s model scenarios utilized real load data, installed capacity, and other inputs whereas the Zero by Fifty study uses forecasts and modeled capacity expansion for 2020 values. This set of model runs also used updated fuel costs from the Energy Information Administration (EIA) 2022 Annual Energy Outlook (AEO) for oil, gas, and coal, with considerably higher prices in the 2020s. The Upper Cost model scenarios further employed middle-of-the-road NREL Annual Technology Baseline capital cost estimates, whereas the Zero by Fifty study considers optimistic NREL ATB future capital cost values.
The model scenarios in this study do not use the distributed energy resources (DER) co-optimization setting, which permits the model to estimate the impact of power plant siting and deployment upon distribution system costs and reconsider the least-cost solution with such considerations in mind. This setting would likely have produced additional system-wide cost savings, but is unlikely to significantly change utility-scale deployment or the overall technology mix of the energy system.
The full report and published findings from the VCE Zero by Fifty study have not yet been released. Consequently, detailed Zero by Fifty results on solar and wind land use, future electricity load assumptions, input cost assumptions, breakdowns of modeled energy system costs, and other topics are not yet available. Upon the release of the full VCE Zero by Fifty study, more extensive comparisons with this analysis will be both possible and valuable.
Climate and Societal Benefits
7. Climate Benefits and Job Creation
Commercializing advanced nuclear technology produces a wide range of potential benefits by mitigating climate change, creating jobs, and reducing energy costs. The modeling results in this report suggest that by 2050, advanced nuclear could provide up to 50 percent of total US electricity demand with clean energy, create more than 223,000 stable and well-paying jobs nationwide, and contribute to increased American energy security and affordability. This section provides quantitative results and a discussion of the potential benefits of advanced nuclear commercialization.
7.1 Climate
The Biden Administration has sought to restore America’s leadership in the global fight against climate change by investing in clean energy. The results illuminate the potential contribution of advanced nuclear power to meeting the Biden Administration’s climate goals.
Upon taking office, President Biden rejoined the Paris Agreement, which seeks to limit the average global temperature rise by 2100 to 1.5 to 2 degrees Celsius above pre-industrial levels. Research published by the Intergovernmental Panel on Climate Change suggests that an unprecedented increase in global nuclear generation may be required, with global nuclear generation increasing to up to 500 percent of current levels across modeled scenarios, to reach ambitious climate targets like 1.5 C at low cost. President Biden has also announced a policy goal of reaching 100% clean electricity in the United States by 2035. Nuclear already accounts for 48 percent of clean electricity generation in the United States at present, and provides a valuable firm source of power to complement the increasing share of variable renewables on the grid. Meeting the administration’s ambitious climate and energy targets will require continued existing nuclear power plant operation, as well as advanced nuclear reactor deployment.
The modeling results, produced with Vibrant Clean Energy, suggest that commercializing advanced nuclear technology could result in rapid growth of clean nuclear generation that would help to meet the administration’s climate goals. The contribution of advanced nuclear to the United States electricity sector in 2050 across the scenarios is summarized in Table 7-1.
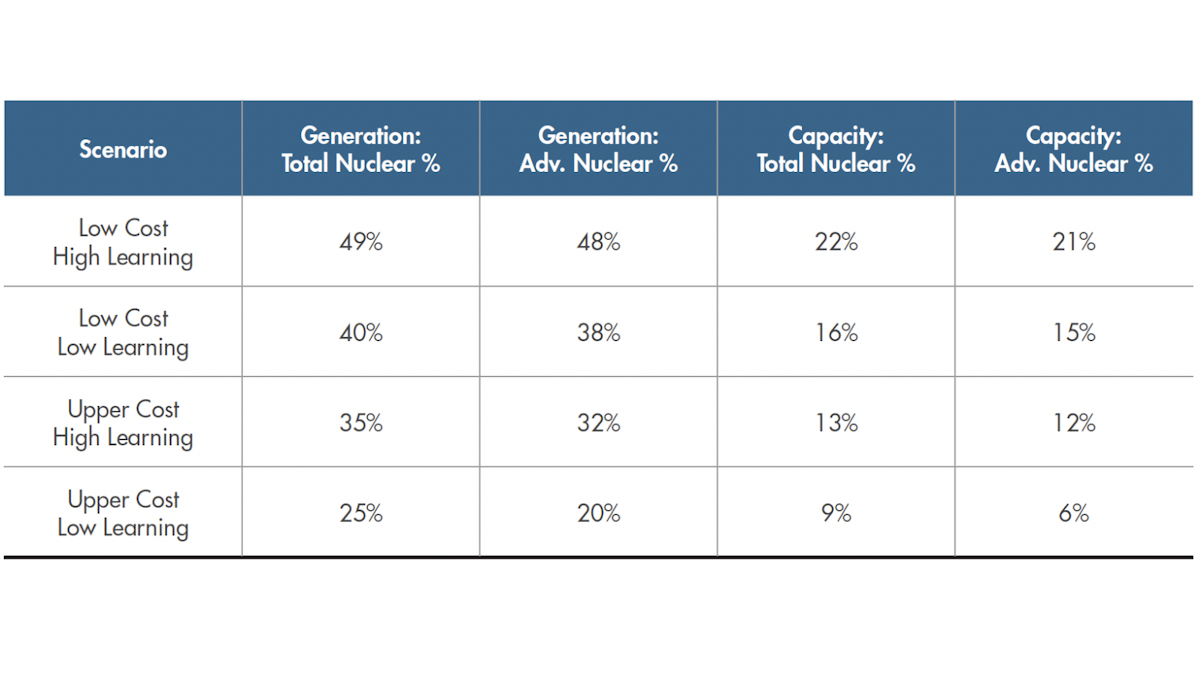
In the optimistic Low-Cost High-Learning scenario, the least-cost pathway to meeting a 2050 net-zero power sector target in the United States would have nuclear power provide approximately 50 percent of the entire US electricity demand, up from 19 percent today.
The majority of this nuclear generation would come from advanced reactors, with the deployment of 469 GWe of advanced nuclear power by 2050. Nuclear energy is able to provide this high share of generation with only 21 percent of the capacity in the electricity system, due to the high capacity factors of nuclear plants relative to other clean sources. Additionally, this growth comes in spite of a steady decline in generation from existing traditional nuclear plants, which declines by 80 percent by 2050 in the Low-Cost High-Learning scenario.
The results illustrate the potential importance of advanced nuclear power relative to solar and wind. In the Low-Cost High-Learning scenario, nuclear generation exceeds solar generation by 75 percent and exceeds wind generation by 50 percent in 2050. This suggests that the market size for advanced reactors could substantially exceed the projected large markets for solar and wind power in the course of achieving a future low-cost net-zero power sector. However, finance and policy support would be necessary to achieve the low costs and high learning rates implied by this optimistic scenario.
In these modeling results, 20 to 50 GWe of advanced nuclear capacity is deployed by 2035. The contribution of advanced nuclear to the United States electricity sector in 2035 is summarized in Table 7-2. Across the scenarios, advanced nuclear power contributes 3 to 8% of US generation by 2035, with all nuclear generation providing 15 to 19% of US generation that year. In 2035, the percentage of total generation from the sum of conventional and advanced nuclear power plants across all scenarios is comparable to generation from wind or solar.
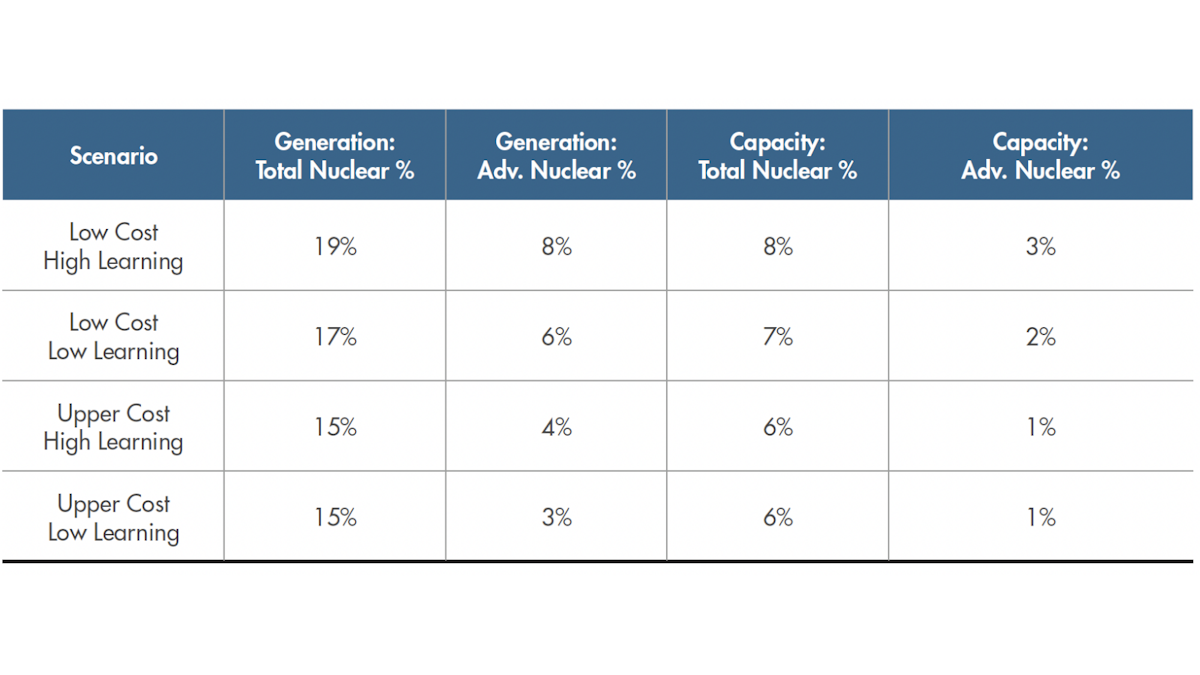
By 2035, the United States achieves around a 60% total reduction of direct power sector CO2 emissions relative to 2020 fossil CO2 emissions across all four of the scenarios. This corresponds to 2035 power sector CO2 emissions of around 700 million metric tons of CO2 (Mt CO2), compared with 2020 emissions of 1,750 Mt CO2. In the model scenarios, power sector emissions fall by 90% relative to 2020 levels by 2045 (175 Mt CO2 in 2045), before the power grid achieves essentially full decarbonization in 2050 (Figure 7-1). Note that the current US grid has already achieved some decarbonization relative to 2010 power sector fossil emissions of 2,400 Mt CO2.
U.S. Power Sector CO2 Emissions
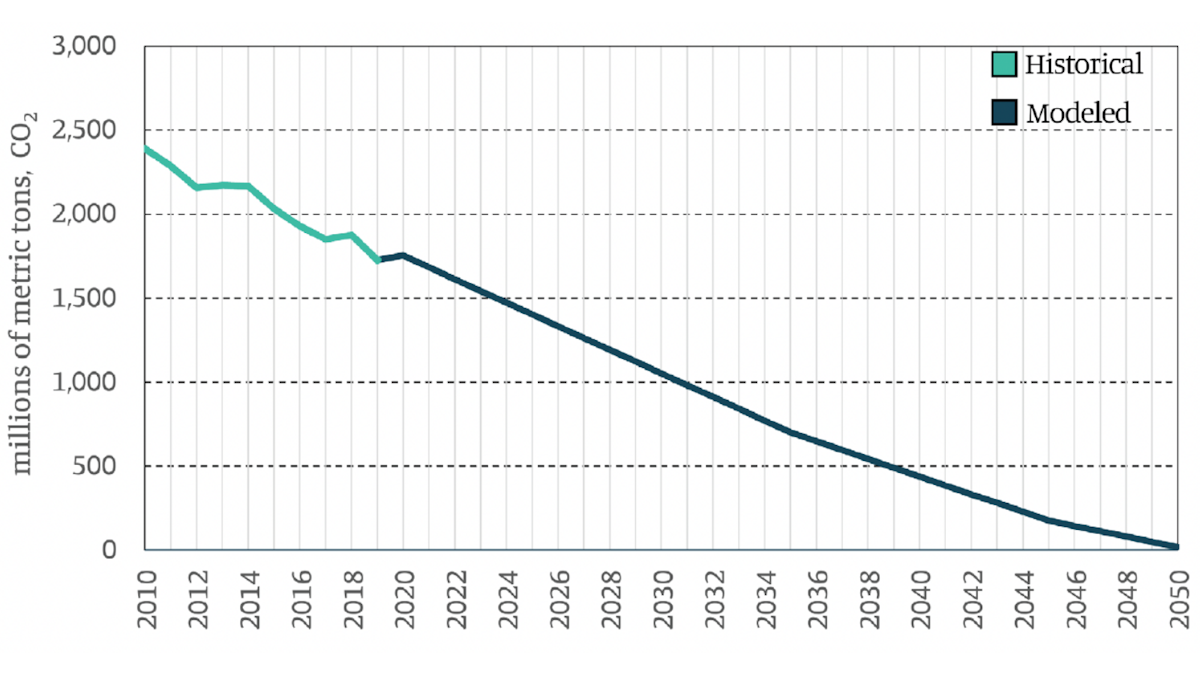
The scenarios used in this report were constructed around a 2050 net-zero power sector target rather than the Biden Administration’s 2035 goal for a zero-emission power sector, which means that these results may understate the potential contribution of advanced nuclear technology in reaching a binding 2035 net-zero target. Reaching a 2035 net-zero target would require substantially more policy and financial support. Across the scenarios, around 70% of the United States generation comes from clean sources in 2035.
7.2 Clean air
Apart from climate benefits via reduced greenhouse gas emissions, the modeled decarbonization pathways for the United States power sector produce dramatic benefits for domestic air pollution. By 2030, the phaseout of coal-fired generation across all model scenarios cuts SO2, PM2.5, and PM10 emissions from the power sector by more than 98%, while NOx emissions fall by around 75% over the same period (Figure 7-2). By 2050, essentially all power sector emissions of criteria pollutants included in the model (CO, SO2, NOx, PM2.5, PM10) have ceased.
As the majority of advanced nuclear power is deployed after 2035, advanced reactors contribute incrementally rather than decisively to the nationwide alleviation of SO2, PM2.5, PM10, and NOx pollution between 2020-2035. Nevertheless, the continued operation of existing traditional nuclear reactors over this period helps avoid the near-term expansion of fossil fuel use. Early advanced nuclear deployment may also support indirect air pollution reductions by enabling more rapid renewables deployment and fossil capacity retirement in certain localities and regions.
Reduction in Power Sector Air Pollution
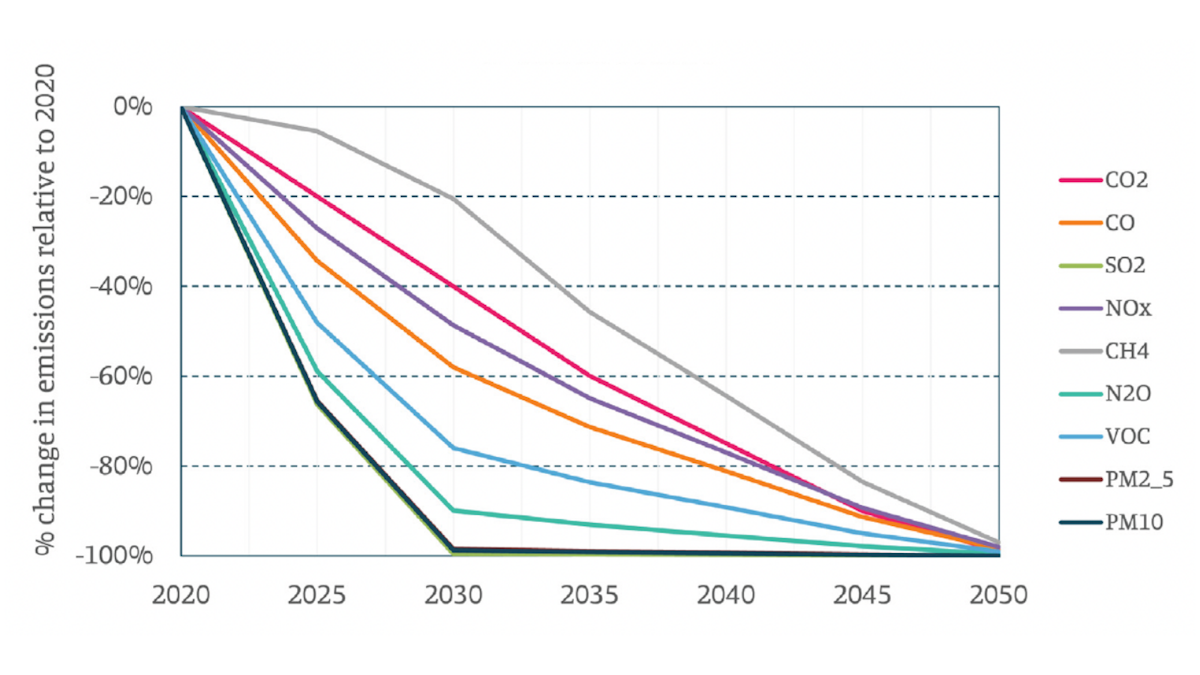
Advanced nuclear energy arguably plays a more direct role in displacing remaining power sector emissions of NOx, CH4, and volatile organic compounds (VOCs). NOx and CH4 pollution from the power sector have both fallen to half of 2020 levels by the 2030s, with VOC emissions declining by 84%. Advanced nuclear reactor deployment alongside other clean energy installations eliminate the remainder of these emissions by 2050. Other pollutants such as ozone are not directly considered by the WIS:dom-P model, but would also experience sharp declines in emissions as a result of power sector and economy-wide decarbonization, as ozone production is driven by other pollutants such as VOCs and NOx.
This transformation of the United States power sector produces considerable public health and environmental benefits. Long-term research by the Clean Air Task Force has estimated that over 3,000 Americans die every year due to the particulate pollution emitted from domestic coal-fired power plants alone. Economy-wide air pollution may contribute to as many as 100,000 to 200,000 excess deaths in the United States annually. Such metrics do not include the even greater impacts of sub-lethal public health risks such as long-term illness and the aggravation of respiratory conditions. Air pollutants can also affect ecosystems and the natural environment, with pollution also affecting sensitive wildlife and with NOx and SO2 contributing to phenomena like acid rain. Power sector decarbonization via a combination of renewables, advanced nuclear energy, and other clean energy sources will not only vastly improve public and environmental health, but will also likely produce substantial national economic benefits thanks to generally improved societal well-being.
7.3 Improved Life Cycle Environmental Impacts
Life Cycle Comparisons Across Clean Electricity Technologies
The true environmental impacts of electricity generation extend well beyond power plant operations. Total life cycle impacts of electricity production include upstream activities such as mining, manufacturing, construction, and the production and shipment of fuels, as well as downstream activities such as waste disposal and materials recycling. Researchers often assess such comprehensive “cradle-to-grave” impacts of electricity technologies using Life Cycle Assessment (LCA) approaches, which quantify climate, environmental, and human health impacts at every step of every process involved in electricity generation, both upstream and downstream.
In contrast to misleading portrayals in popular mass media, nuclear energy produces fewer environmental and public health impacts than most other forms of energy production. The total life cycle impacts of electricity generated from nuclear power plants are often similar if not improved relative to wind or solar electricity. Consequently, a future US power sector featuring a sizable fraction of advanced nuclear generation alongside wind and solar technologies will likely represent a vast improvement over the fossil-heavy power sector of today.
The United Nations Economic Commission for Europe (UNECE) recently published a major report analyzing a broad suite of life cycle impacts for a wide range of electricity generation technologies, including nuclear power. In terms of life cycle greenhouse gas emissions, nuclear energy was the lowest-emitting source of electricity examined (range of 4.9 to 6.3 g CO2 emitted per kWh of electricity generation), well below that of even utility-scale solar PV (23 to 82 g CO2/KWh) and even slightly below calculated emissions for onshore wind (7.8 to 16 g CO2/KWh). For most other life cycle impacts such as freshwater eutrophication, human toxicity, land occupation, mineral and metal requirements, and life cycle fossil fuel usage, impacts associated with nuclear power were scored as comparable or better than other clean energy technologies such as renewables. The UNECE similarly rated nuclear energy as equal to or superior to wind and solar generation when considering overall life cycle ecosystem impacts and overall life cycle human health impacts as a whole.
Nuclear energy results in markedly lower life cycle environmental impacts than competing clean energy technologies like wind and solar in two categories in particular: land use and mineral/metal requirements. Nuclear power plants produce large quantities of electricity and heat relative to the land footprint they occupy, on the order of 240 watts of generating capacity per square meter. This is two orders of magnitude more land-efficient than utility-scale solar PV installations (4-8 We/m2) and onshore wind power (2-3 We/m2).
At the same time, nuclear reactors require fewer minerals and metals than solar and wind installations on both a per-unit-capacity and per-unit-generation basis. Lower mineral requirements in turn translate into lower upstream land-use impacts from mining and mineral processing. Power sector decarbonization pathways that are able to leverage more materials-efficient generation sources like nuclear can thus significantly reduce not only overall material demand but also the environmental impacts associated with materials sourcing.
Uranium Mining
Modern uranium mining practices have improved significantly relative to practices half a century ago, thanks to the adoption of better mining techniques and stronger oversight and accountability frameworks, including public and tribal engagement. Starting in the 1980s, a technique for uranium extraction called in-situ recovery (ISR) made it possible to mine uranium without pit or shaft mining altogether and has become increasingly widespread. ISU works by leaching uranium directly from the ground using a chemical extraction method, which poses less disturbance to the environment than pit or shaft mining. In 2019, over half of globally-mined uranium was produced via ISR. Nevertheless, there are potential impacts that require monitoring to ensure safety, such as groundwater testing.
Historically, irresponsible uranium mining practices and inadequate environmental and labor safeguards exposed workers and communities to radiation, particularly at the height of the Cold War during the peak of nuclear weapons production. Negligent mining left and continues to leave impacts that have particularly harmed Indigenous peoples whose lands were often targeted for uranium ore production, including Navajo and Hopi people in the United States, Aboriginal Australian communities, and Indigenous Tribes in Northern Canada. Many legacy uranium mine sites sit abandoned, with mines and mine tailings having remained un-remediated in some cases for generations, posing continued hazards to local communities today.
To address US indigenous tribes, the NRC engages in extensive interactions with every tribe in the United States that is located near mines, and other nuclear facilities. As US interest in advanced reactors may lead the United States to restart operations at idled uranium mines, regulators, the uranium industry, and downstream nuclear customers should all continue to enforce regulations and best practices that minimize impacts to the environment and local community. the United States federal government must furthermore fulfill long-unmet obligations to communities impacted by uranium mining by allocating additional funding and resources to accelerate remediation of abandoned mines and DOE legacy sites.
Spent Fuel and High-Level Nuclear Waste
Uncertainty regarding the disposition of spent nuclear fuel remains a significant source of opposition to nuclear energy from politicians and the public. the United States federal government has a self-imposed legal obligation to take title to spent fuel from commercial reactors and deposit it in a geological repository at Yucca Mountain, Nevada. The federal Nuclear Waste Fund, which was collected from utilities to pay for the development of a repository, currently holds approximately $45 billion. However, since progress on the Yucca Mountain site was halted and defunded during the Obama administration, the federal government has been in violation of its own mandate, and has had to pay over $7.5 billion back to nuclear power companies in lawsuits, and collection of nuclear waste fund fees has been paused. The fund continues to collect interest and grow by $1.5B/year. While US companies are currently doing an excellent job managing spent fuel, the United States needs to establish better long-term solutions. In short, nuclear waste management remains a significant issue for nuclear policy, but not safety, in the United States
The outlook for nuclear waste management shows positive signs. First, the current practices for spent fuel management at reactor sites, especially storing spent fuel rods in large, thick concrete dry casks, has an excellent safety record, with zero release of harmful radiation to the public in decades of operation. Second, innovative waste management technologies, like deep borehole drilling, offer new opportunities for spent fuel management that may not require the construction of a large central mined repository like that which was planned for Yucca Mountain. Third, international peers like Finland have made substantial recent progress toward developing centralized nuclear waste repositories, with the deep geological mined repository at Onkalo, Finland, substantially constructed and scheduled to commence operation in 2023. That is in contrast to France, which has close the fuel cycle by reprocessing of spent fuel a core policy.
In a future with high levels of nuclear deployment and/or geopolitical constraints on nuclear fuel supply (e.g. from Russia and Russian allies), nuclear waste could become a valuable source of fuel for advanced reactors. This is already the case in France, which has reduced demand for natural uranium by almost 20% from reprocessing spent fuel.
The increased efficiency and optimized design of advanced reactors may result in less nuclear waste produced from operation relative to older designs. Several advanced nuclear companies have offered reactor designs that could run on spent fuel with minimal reprocessing, including General Electric’s PRISM, Moltex, and Oklo. The ARPA-E Optimizing Nuclear Waste and Advanced Reactor Disposal Systems program seeks breakthrough technologies to facilitate a 10x reduction in waste volume with back-end costs in the range of $1/megawatt-hour.
7.4 Jobs
Nuclear energy can play a positive, central role in the economic livelihood of local communities where nuclear power plants are based. Today, the nuclear power sector supports nearly 500,000 jobs across the United States, which can pay 30% higher than the local average. , Important community and public revenue is generated through local, state and federal taxes which can amount up to $30 million per plant just at the local level. Consequently, communities around nuclear facilities are often wealthier, with higher incomes and increased property values. Advanced reactors possess a similar potential to grow local employment, economic activity, and public capacity just as the nuclear industry has done to date.
Operations and maintenance jobs
Across the four models, the estimated range of operations and maintenance jobs at advanced nuclear power plants (Figure 7-3):
- 2035: 8,000 to 22,000 permanent jobs
- 2040: 22,000 to 654,000 permanent jobs
- 2050: 74,000 to 223,000 permanent jobs
Advanced Nuclear Permanent O+M Jobs
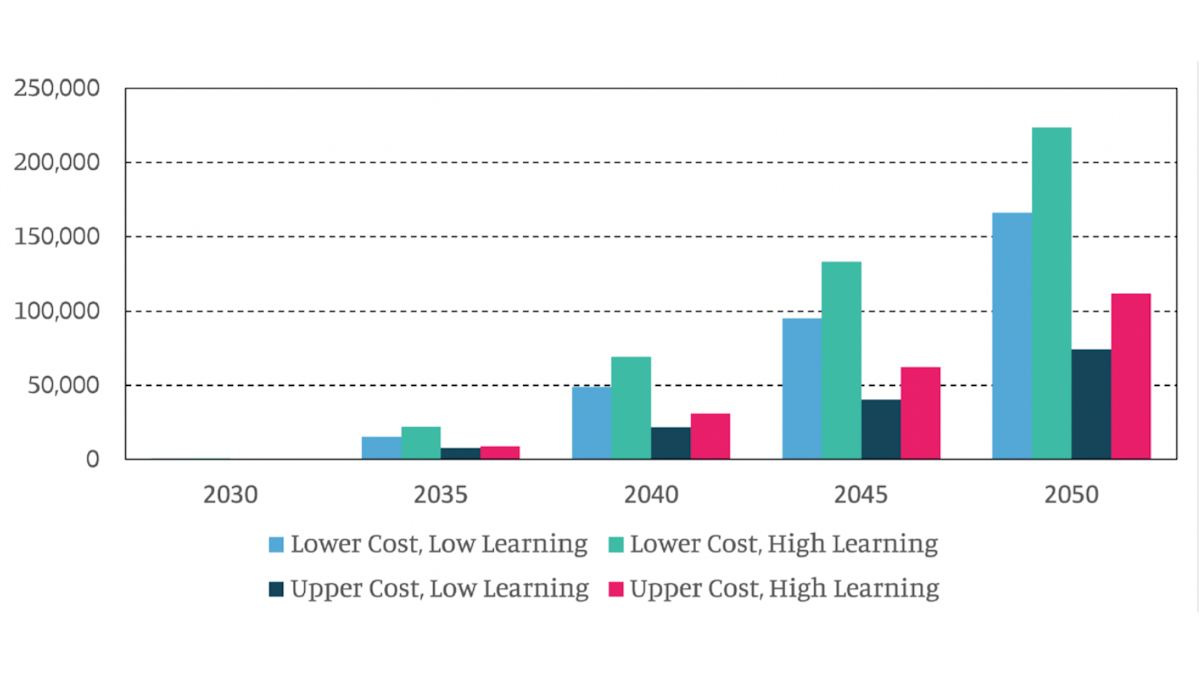
Operations and maintenance jobs are estimated based on installed advanced nuclear capacity and reactor types operating in that year, multiplied by employment factors determined from a range of published literature as well as projections provided by advanced nuclear vendors under confidentiality agreements. This category considers onsite, full-time power plant staff only.
By 2050, up to a couple hundred thousand Americans are directly and permanently employed at advanced nuclear power plants in operations and maintenance positions. This is as much as double the 100,000 direct permanent jobs supported by US nuclear power plants today. In combination with manufacturing, construction, indirect, and induced employment, it is likely that a successful future US advanced nuclear sector could support significantly more jobs and economic activity than the already-large US nuclear industry does today.
Manufacturing Jobs
A 2004 INL study suggested that at least 37,000 manufacturing jobs could be repatriated to the United States-based on government incentives and a hypothesized buildout of 50 GWe of traditional nuclear power plants in the United States. Using this old estimate as a basis for general discussion, potential factors that could determine how domestic employment within a future advanced manufacturing sector are qualitatively assessed. Direct estimates of advanced nuclear manufacturing jobs are difficult to predict, given large uncertainties today about the future manufacturing models that vendors will pursue to mass-produce advanced reactors.
- Demand: It is anticipated that demand for advanced nuclear reactors will be both domestic and international, with a substantial fraction of the demand being international.
- Number of Jobs Repatriated: The INL study assumed that all manufacturing jobs would be repatriated jobs. This study refers to repatriated jobs as those which were previously associated with internationally-sourced procurements, and which the United States will have the capacity and capability to supply for advanced reactors. Hence, for this study, using the Westinghouse AP1000 procurement approach, the major components that were sourced internationally included steam generators, reactor vessels, head assemblies, and the turbine generator. Thus, approximately half of the other components that make up the primary and secondary systems are domestically available, which include components such as the reactor coolant pump, major valves, pressurizers etc. Therefore only approximately half of the major components are considered as contributing to domestic employment thanks to repatriated jobs.
- Considerations for Localization of Supply Chain for International Orders: international customers of US nuclear technology will likely be interested in sourcing components locally to the extent possible to support local economic development. Hence, for meeting international advanced nuclear export opportunities, some components that can be procured in the United States will be procured internationally.
- Considerations for Smaller Scale Components: The INL study assumed all major components would continue to be sourced internationally because the reactors in consideration in the study were large plants such as the AP1000, which require large forgings that are only available from a few international suppliers. However, reducing the component size by up to 20% exponentially increases the number of suppliers both internationally and domestically that can provide the components. Hence, for small modular reactors and microreactors, it is anticipated that more major components for these plants can be locally sourced in the United States.
Plant Construction Jobs
The 2004 INL study assumed 72,000 construction jobs would be created by a 50 GWe buildout of conventional nuclear capacity. A large-scale advanced nuclear buildout throughout the United States would differ from this theorized large light-water reactor construction program in several ways:
- Increased Manufacturing and Factory-Based Scope Due to Modularization and Technological Improvements: It is expected that small modular reactors and microreactors will be largely manufactured in factories, with minimal on-site scope. In addition, factory manufacturing techniques have progressed markedly since the INL study’s publication due to technological progress in areas like robotics and logistics. Hence, it is expected that a successful domestic advanced nuclear industry will create fewer construction jobs than what the INL study assumed.
- Reduced Site Development Scope: Large reactors require a significant amount of civil works associated with items such as deep excavations, reinforced foundations, and transmission infrastructure, which would be of a substantially smaller scale for small modular reactors and microreactors. Hence, the civil and site construction scope and associated jobs would be significantly less.
- Accelerated and Standardized Construction Techniques: Due to the modular, standardized nature of many advanced reactors, it is anticipated that advanced nuclear power plant construction will adopt similar if not identical power plant designs, leading to faster learning-by-doing and encouraging an industry construction model in which smaller construction crews move from one project site to the next project site and complete builds more rapidly. Such factors might further reduce construction personnel requirements per unit of advanced nuclear generation capacity.
Given these considerations in conjunction with uncertainties involved in distinguishing between permanent and temporary construction jobs across both concurrent and sequential small reactor projects over the length of a 30-year study period, construction jobs created by a successful US advanced nuclear sector are not estimated. However, the modeled buildout of advanced nuclear reactors nationwide reaches 190 GWe to 470 GWe of operational capacity by 2050, a buildout that is four to nine times the size of the conventional nuclear construction program envisioned by the INL study. It is therefore conceivable that the future US advanced nuclear sector could employ a couple hundred thousand Americans in advanced nuclear power plant construction by the 2040s.
Indirect and Induced Advanced Nuclear Jobs
The 2004 INL study assumed that 181,000 indirect jobs would be created for a cumulative nationwide installation of 50 GWe of conventional nuclear capacity. In contrast, SMRs and microreactors are expected to be deployed in more locations than assumed for the large plants in the INL study, which considered a reactor unit capacity of 1200 MWe and envisioned the construction of 41 plants. Advanced nuclear reactor unit capacities of 80 MWe to 350 MWe enable far more spatially extensive deployment of advanced nuclear power plants even considering the installation of multiple units at the same site, potentially generating increased nationwide indirect economic benefits and job opportunities. However, the advanced nuclear supply chain will differ sufficiently from the traditional nuclear industry to introduce large uncertainties into an assessment of indirect job creation potential.
Induced jobs are created outside the nuclear industry, but are required to support the industry and its workers. These include jobs that support additional infrastructure and amenities built because of the nuclear power plant, such as grocery stores, schools, restaurants, etc... In the case of advanced reactors, a variety of factors may increase, decrease, or not affect estimates of induced job creation relative to conventional nuclear plants. The smaller scale of advanced nuclear plants may result in a more limited increase in local services and associated workers. As with indirect jobs, the secondary economic effects of advanced nuclear construction are difficult to evaluate. Nevertheless, considering the large scale of advanced nuclear capacity installed in the model over the next three decades, the total indirect and induced job creation potential may prove considerable.
7.6 Just Transition
A just transition refers to a shift in the energy landscape from fossil fuels to clean, zero-carbon energy technologies that avoid leaving workers in the fossil fuel, automotive, and other sectors stranded with no opportunities to adapt or adjust to these changes. Nuclear energy offers unique advantages for helping enable a just transition given its versatile co-benefits outside of electrical production. This may allow a wide range of fossil fuel workers to transfer their relevant skill sets to new but related areas of employment within a decarbonized US economy.
Beyond the power sector, the potential of advanced nuclear reactors to support the co-generation of non-electric products such as hydrogen or synthetic fuels may provide additional opportunities to support a transition away from fossil fuels, as both the future clean hydrogen sector and the supply chains needed to refine and transport synthetic fuels will leverage additional components of the current fossil fuel sector.
Many fossil fuel plants, including coal plants, are already attractive for nuclear reactor siting since they are already located near cooling water sources, integrated within transmission networks, and safely distanced from local populations. Retrofitting coal plants for nuclear energy also directly allows for re-employment of workers and economic support of communities impacted by the coal plants’ closures.
Exploratory efforts to deploy next-generation nuclear projects at retiring fossil fuel plants are already underway. The Natrium advanced nuclear power plant is set to be built by TerraPower over the next 7 years in Kemmerer, Wyoming, replacing the coal-powered Naughton plant after its retirement in 2025. The project has generated strong community support from local Wyoming residents, who value the project’s potential to transition thousands of local coal power plant workers to construct, operate and maintain the nuclear plant. The Kemmerer project illustrates how advanced nuclear energy can enable an economically advantageous transition away from the fossil fuel industry for communities that previously depended upon fossil generation. Siting advanced nuclear reactors at decommissioned coal-fired power plants can harness the available infrastructure and expertise that already exists in these communities while developing the United States advanced nuclear sector over the long-term.
Fossil-to-Nuclear and Nuclear-to-Nuclear Repowering
The results highlight a sizable potential for advanced nuclear projects built at the same site as fossil fuel and conventional nuclear power plants currently operating today (Figure 7-4). Combined with forward-thinking public policy, advanced nuclear deployment at existing power plant sites could enable project cost savings through the reuse of existing transmission, cooling, steam-cycle, and balance-of-plant infrastructure while generating local community benefits through job creation that replaces lost employment from retiring fossil or nuclear plants.
The WIS:dom-P model takes several factors into account when siting new power plants, such as existing transmission networks, the availability of a cooling water supply, the prioritization of brownfield over greenfield development, and more. As such, this result suggests strong cost-optimization reasons for deploying new nuclear projects at existing fossil fuel and nuclear energy generation stations. Over the next three decades, the scenarios lead to the deployment of advanced reactors at 146 to 254 existing power plant sites nationwide. These projects are largely built after 2035, with most builds occurring in the 2040s. Apart from hard-coded advanced reactor demonstration projects, deployment of new nuclear capacity prior to 2028 was not permitted in the model.
Site Conversions to Advanced Nuclear Across Scenarios
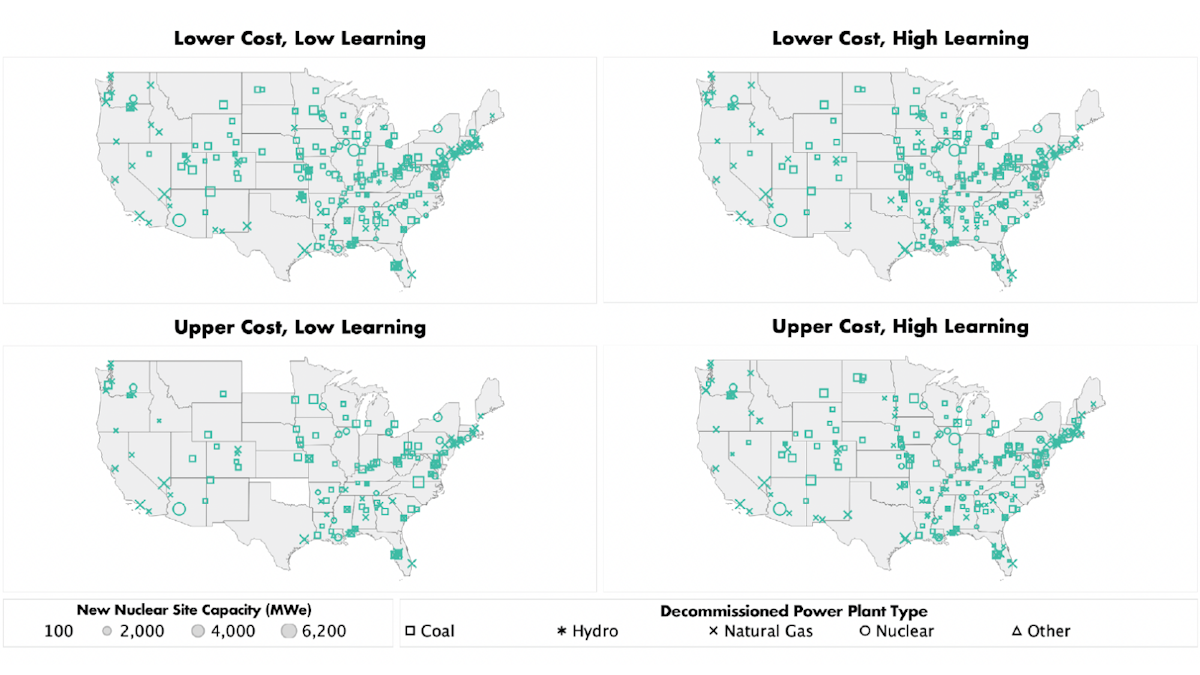
The model deploys advanced nuclear at existing coal-fired power plants that total between 109 and 153 GWe of coal generating capacity, equivalent to 52-73% of the domestic coal power capacity today. The existing natural gas power plants where new nuclear units are built account for between 34 and 71 GWe of current gas generating capacity, or 7-14% of the gas-fired power sector. New nuclear units are also built at current nuclear power plants with a combined 31 to 63 GWe of operational capacity today, which corresponds to one to two-thirds of the 95 GWe US conventional nuclear capacity at present. In total, new advanced nuclear projects are deployed at between 130 to 223 currently-operating fossil-fired power plants (Figure 7-5).
Number of Advanced Nuclear Site Conversions and Average Duration Between Power Plant Decomissioning and Site Convertion Across Scenarios
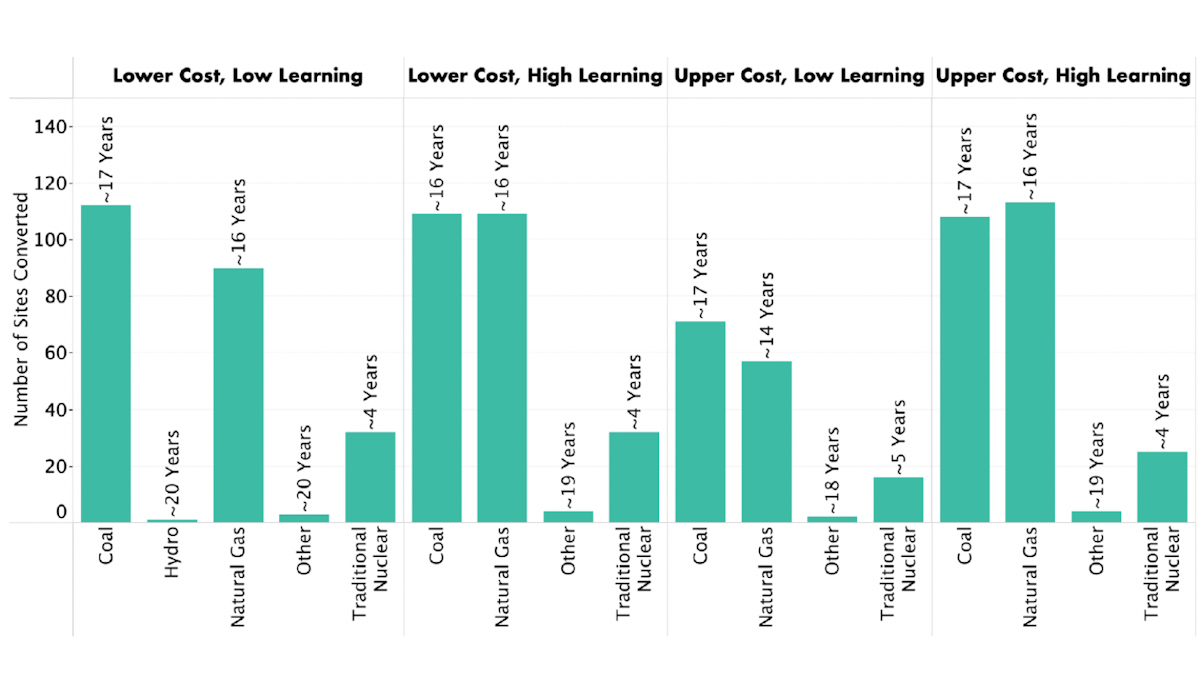
In most cases, the modeled decommissioning of the existing power plant and the commissioning of new advanced nuclear capacity at the same site are separated by a considerable temporal lag of a decade or more. The average modeled site conversion delay time for fossil-fired power plant site conversions is 16 years, noticeably higher than an average of four years for traditional nuclear plants. Importantly these averages are over five-year time periods. In other words, an average below five years may be considered to be in the same time period. The Vibrant model makes no effort to minimize the delay between site decommissioning and advanced nuclear conversion.
The observed lag between re-powering fossil-fired power plants using a least-cost model emphasizes the importance of proactive planning should policymakers and stakeholders wish to maximize fossil power plant job retention and site equipment reuse (see recommendations in Section 9.2). A prolonged period of even just a few years between the decommissioning of a fossil-fired or traditional nuclear power plant and the start of advanced nuclear construction will substantially reduce the potential for re-employment of existing plant workers and reuse of infrastructure. Planners should endeavor to make all necessary preparations for a new nuclear power project years, if not a decade, in advance of projected fossil plant retirement.
At the same time, the observed temporal lag suggests that the results represent a somewhat optimistic assessment of the potential for repowering of existing coal and gas capacity with advanced nuclear power. The model meets climate targets by decommissioning substantial coal and to a lesser extent gas capacity in the 2020s and early 2030s, whereas the deployment of advanced nuclear projects does not accelerate at a nationwide scale until after 2035. This finding suggests that climate goals will drive fossil plant retirements on nearer-term timescales that make plans to repower decommissioned capacity with advanced nuclear power more difficult. On the other hand, pursuit of faster power sector decarbonization with a target date earlier than 2050 could change this calculus and necessitate more near-term advanced nuclear builds.
The modeled potential for advanced nuclear builds at existing conventional nuclear sites, however, may be more realistic. Conventional nuclear power plants that host new advanced nuclear projects in the model scenarios typically see existing conventional reactor units decommissioned in around the same timeframe that the advanced reactors enter operation. This suggests a stronger potential for contemporaneous repowering of today’s nuclear facilities with advanced nuclear technology in the near term, enabling more ambitious efforts to retain nuclear plant workers and re-use existing plant infrastructure.
The Path Forward for Building a Successful Advanced Nuclear Sector
8. Barriers to Nuclear Power Development and Deployment
The most important barriers to greater cost-effectiveness and economic competitiveness of nuclear power deployment are created by the following factors: technology complexity, the scale of the components and facilities, and a slow and uncertain regulatory licensing process that is not designed to facilitate innovation. These major categories of issues are interdependent and create a compounding effect that drives cost.
Historically, the need to optimize power output increased the size and scale of the plant components, which increased complexity because of the need to include additional components to ensure the efficient and safe operation of larger sized components. An example of this is the need for an active and mechanically operated reactor coolant system for large plants, which is not necessary for certain small modular reactor designs, since they can achieve passive cooling, and require no special equipment. Additional components in a mechanically operated coolant system created more potential points of failure, which increased technical scopes related to analysis, modeling, validation, equipment and system qualification, and other areas. This increased system complexity further drove additional regulatory standards, scrutiny, and requirements. The larger sizes also significantly affected the supply chain by creating oligopolies, as only certain suppliers could provide large nuclear components. The time required to develop and deploy these larger systems also increased with reactor size.
8.1 Supply Chain Barriers
While advanced reactor designs may be able to benefit in the future from inherent advantages that simplify supply chain considerations, the component manufacturing pipeline could present a challenging bottleneck over the next decade. In particular, the current US-based supply chain has not been developed to support future advanced reactor deployment. Multiple issues, both strategic and capacity-related, would need to be addressed. The strategic elements include preventing future oligopolies, supporting domestic job creation, managing obsolescence, and ensuring nuclear security. Capacity issues include providing the infrastructure to manufacture major components domestically or in close partner countries at a sufficient scale, including providing large forgings, specialized alloys, and fuels.
Issues Related to Scale
Cost estimations for advanced nuclear power plants should factor in the issues related to scale and complexity and perform the trade-off analysis relative to higher power generation, to ensure that the final costs on a dollar per megawatt basis are competitive. This assessment should drive plant sizing, which would in certain cases lead to investment decisions on small modular reactors and microreactors. The potential benefits of smaller reactors will include more applications and deployment scenarios. For instance, developing nations may benefit from greater distributed generation due to less robust transmission and distribution infrastructure.
Issues Related to Cost-Effectiveness
Increased competition will help to drive down cost. Hence, oligopolies should be prevented in future advanced reactor supply chains. Oligopolies can be prevented by encouraging smaller designs that can be built by several vendors. Another useful measure will be the continued provision of access to critical government technologies and technical experts in the United States national laboratory complex through programs such as the Gateway for Accelerated Innovation in Nuclear (GAIN).
Issues Related to Capabilities and Capacity
To maximize job creation opportunities and support the anticipated demand for new plant construction, domestic nuclear supply chain capacities and capabilities must be enhanced. Key factors include the ability to produce major components, such as the manufacturing and fabrication infrastructure within the AP1000 module fabrication facility. Supply chain capabilities also include the knowledge and qualifications to produce nuclear grade components, such as nuclear grade quality assurance programs including NQA-1 certifications. The federal government should invest in the development of strategic nuclear fabrication infrastructure, which should be developed in a manner that can support multiple reactor types and facilities. the United States government should also invest in capability development to ensure that personnel, processes, and tools are prepared to produce nuclear quality grade components, by supporting other widely accepted quality assurance programs like ISO:9001.
For advanced nuclear designs, considerable variation may exist between supply chains for different nuclear reactor technologies. These technical differences may complicate efforts to standardize components in upstream supply chains in ways that meet the needs of multiple developers. For instance, advanced reactors that are cooled using water, molten salt, or helium gas will require cooling system parts with different specifications to handle the particular needs of these distinct cooling mediums. Similarly, steam cycle equipment will be designed based on the operating characteristics of designs with different outlet steam temperatures and reactor/generator sizes.
Fuel types will also vary among the different advanced reactor designs. For instance, the Natrium sodium-cooled fast reactor design under development by TerraPower or the Xe-100 high-temperature gas-cooled pebble-bed reactor designed by X-Energy may utilize TRi-structural ISOtropic (TRISO) particle fuel enriched to a high-assay, low-enriched uranium (HALEU) standard. In contrast, the GE-Hitachi BWRX-300 or NuScale small modular reactors will use more conventional fuel pellets and fuel rods.
To meet future supply chain requirements, the public sector and industry stakeholders should work together to proactively develop and expand manufacturing pipelines (for inputs and components) that do not currently exist. Agreements between nuclear industry actors and suppliers such as the recently-announced partnership between NuScale and the Korean industrial firm Doosan to secure forged reactor components will also play a crucial role in bolstering the advanced nuclear supply chain. Such consortia can help coordinate the business strategy of small suppliers or formalize the involvement of larger suppliers that can play key roles in guaranteeing access and availability.
Where possible, industry actors and researchers should exploit opportunities to share information and standardize components and parts across designs to take advantage of supply chain simplification and manufacturing economies of scale. Advance pre-orders by utility and private customers and public sector procurement of reactor projects can also help demonstrate demand for nuclear supply chains and incentivize accelerated industry development. Some of these results are addressed in the subsequent major report section on policy incentives and policy support (see Section 9).
8.2 Regulatory Barriers
The only advanced reactor to receive a design certification from the NRC to date is the 60MW version of the NuScale power module. This design certification took approximately five years and cost $70 million in fees to the NRC. NuScale will need to secure a site license in addition to the design certification and resolve open issues before the first power plant can be constructed. Despite this initial investment, NuScale plans to move away from this 60 MW version to a more powerful 77 MW version.
Advanced reactor developers and licensees are challenged by the fact that the current regulatory regime was developed for existing conventional large light-water reactor designs. The licensing framework is written in a prescriptive manner, which makes it difficult for advanced reactors with different parameters to efficiently progress through the licensing process.
To address this issue, the United States Congress enacted the Nuclear Energy Innovation and Modernization Act of 2018, which directed the NRC to develop a regulatory framework for optional use by licensees. The NRC has committed to complete this revamped regulatory framework, which is designated as Part 53, in 2025. This regulatory framework includes the following components:
- Technology-Inclusive: This approach allows the regulation to consider all technologies, instead of being designed for a specific technology class. This means the regulation could be applied equally to SMR, HTGR, ARTES, and other technologies.
- Performance-Based: This approach allows the licensee to meet the regulatory objectives by methods of their choice for demonstrating compliance. This is different from a prescriptive approach that specifies ‘how’ requirements should be accomplished. The performance-based approach is focused on ‘what’ must be achieved. This allows the licensee to be innovative and cost-effective in its approach.
- Risk-Informed: This includes a quantitative assessment of risk to identify the most important risks to focus on and allows for appropriate levels of regulatory action relative to the risk. With this approach, the scale and size of SMRs would allow for adjustment of regulatory requirements to account for the smaller risk profile. An example of this is the emergency planning zone around the plant — the area that requires a special plan in the event of an accident. A risk-informed approach would allow the SMRs to have a smaller area than what would be required for a large plant that poses a larger risk because of a larger fuel inventory.
With the Part 53 framework still under development, the priorities for overcoming regulatory obstacles to advanced reactor deployment are twofold. First, the federal government must facilitate ongoing efforts by early advanced nuclear developers to navigate licensing and project approval processes using currently-existing, and constantly changing, licensing regulatory pathways. Second, the NRC must complete the modernized Part 53 regulatory code on-schedule, and the new framework must allow for timely, efficient review and licensing of advanced nuclear designs and proposed new nuclear projects.
Given that an updated Part 53 framework does not yet exist, prospective advanced nuclear vendors are currently striving to license their designs via existing regulations that were designed to apply to large, conventional, light-water nuclear reactors. The substantial technical differences between conventional nuclear designs and advanced designs have led developers to adopt improvised approaches to secure licensing approval using current regulatory pathways. For instance, advanced nuclear vendors have had to seek exemptions for certain systems or infrastructure that advanced nuclear designs do not require. Many advanced reactors contain smaller quantities of nuclear fuel, feature inherent safety characteristics like passive cooling systems and highly-resilient fuel, and utilize more streamlined, mechanically simplified operating principles. However, the process of obtaining numerous exemptions and meeting regulatory standards that were written with conventional reactors in mind has prolonged the licensing timeline for advanced nuclear developers, increasing costs and making it more difficult for vendors to secure private investment. Nonetheless more than a dozen advanced reactor developers are pursuing NRC licensing and attracting private investment.
The lengthy duration of NRC licensing reviews could slow deployment of advanced nuclear reactors. Historically, a license review for a complex large LWR often took five years or more to conduct. Even with a well-prepared application the NRC estimates that licensing under existing frameworks will take about three to four years using 10 CFR Part 52, and six to seven years to complete both phases under 10 CFR Part 50. However, advanced reactors are expected to be simpler, smaller, and safer than LWRs. A licensing review timeline of only one ot three years will be needed to facilitate business models that reduce cost and allow for the level of deployment found in this report. Unnecessarily long licensing reviews can raise significant barriers to investment, reduce customer interest in advanced reactors, and threaten their successful long-term deployment.
The funding structure for the NRC inhibits advanced nuclear innovation by reducing operational flexibility and efficiency and imposing a significant barrier to new entrants in the form of upfront licensing costs. The NRC’s budget has declined more than 30 percent since the mid-2010s due to plant retirements and reduced application activity, accompanied by a 25 percent reduction in NRC staff. The NRC receives fees almost entirely through direct charges to licensees, with relatively little funding from the federal government. This constrains the NRC’s ability to conduct broad, important rulemaking, licensing reviews, and proactive research to support risk-informed, performance-based regulation, and assign and prepare staff ahead of application submittals. License applicants must pay millions of dollars in NRC fees years before they begin earning revenues. This is particularly burdensome for developers with limited capital and new customer types.
Proactive, priority efforts by the NRC with the support of the federal government can streamline efforts to license advanced nuclear designs using existing regulatory frameworks. NRC leadership and staff will need to consider academic, industry, and expert feedback and adopt more flexible, open-minded policies and procedures to avoid imposing unnecessary or non-applicable requirements on advanced nuclear developers. An institution-wide shift that more holistically considers the societal and environmental benefits of nuclear energy alongside managing risks and ensuring robust oversight and accountability will also improve the NRC’s approach to advanced nuclear licensing. At the same time, the nuclear energy industry will play an important role in improving the licensing process by providing input on policies and activities and by promoting efficiency as participants in the regulatory process.
In the future, it will be crucial that the new Part 53 framework for licensing advanced reactors adopt a new, innovative approach to nuclear power regulation rather than simply duplicating existing regulatory rules and guidelines. This regulatory system will need to prioritize prompt, efficient timelines for design and operating license review, in anticipation of an energy system future where dozens of designs and potentially hundreds of proposed projects may be seeking approval simultaneously. A lengthy, complicated, or excessively uncertain regulatory system will directly increase the cost of advanced nuclear deployment, artificially constraining the rate at which advanced reactors can enter widespread commercial service.
8.3 Spent Nuclear Fuel and Waste
At present, the total spent fuel and high-level nuclear waste produced domestically from nuclear power is minimal, and can be safely and efficiently managed via long-term on-site storage in secure concrete dry casks. However, in anticipation of a future in which domestic advanced nuclear energy production may expand considerably, policymakers should pursue additional spent fuel management strategies. Developing sound and efficient regulatory and permitting frameworks for a diverse array of spent fuel management options will make such services more affordable while increasing public support for nuclear technology. Federal agencies should continue to develop a robust national consent-based siting process for spent fuel storage facilities and other back-end fuel cycle services such as vitrification or deep geologic disposal.
Another option for spent fuel management is recycling, which significantly reduces the quantity of waste in need of long-term storage while producing new useful fuel for domestic reactors. Recycling of spent nuclear fuels is practiced in several countries, such as France, but is not currently practiced in the United States This is in part due to prior policy intended to prevent proliferation and the lack of a financial incentive. Despite increasing fuel costs to a small extent, France prioritizes reprocessing as a way to reduce the volume of spent fuel by up to 96 percent while contributing to the country’s energy independence by reducing uranium consumption.
Recycling may ultimately require the NRC to resume rulemaking efforts to develop regulatory frameworks for the licensing, construction, and operation of spent fuel recycling facilities in the United States. The NRC and other parts of the federal government should remain attentive to ongoing trends in the back-end nuclear fuel cycle and to new technical developments in reprocessing and recycling capabilities globally, in order to react efficiently to technological changes and shifts in global demand for such services.
8.4 Project Management
Poor management of nuclear construction projects has historically been a major source of cost escalation and increases in build time. Delays and cost overruns have resulted from the need to make design changes mid-construction, from the turnover of contractors, and from redoing initial construction that failed to meet standards.
Delays directly contribute to cost overruns by lengthening the period over which interest on initial capital costs accumulates. As such, effective planning and project management can facilitate the deployment of new advanced reactors by bringing down initial project costs, enabling more efficient construction, and boosting the confidence of investors and customers by demonstrating on-time, on-budget delivery.
Measures to improve nuclear power plant project management include full completion of engineering design and regulatory licensing prior to the start of construction, better supply chain integration, and efforts to reduce subcontracting. Quality control and technical expectations should be reinforced in advance of each phase of work and then enforced throughout construction. Advanced reactors may also be able to benefit from inherent advantages of greater modularity, such as standardization of power plant infrastructure and layout, or long-term partnerships with suppliers to ensure high-quality component manufacturing.
8.5 Workforce
Growth of a skilled workforce will need to accompany a growing domestic advanced nuclear sector. Critically, efforts to attract, educate, and train nuclear sector workers must take place prior to and alongside the initial stage of advanced reactor deployment. Otherwise, a shortage of operators, technicians, and engineers throughout the nuclear sector from fuel manufacturing to plant operations may constrain the pace at which new nuclear capacity can enter service.
Successfully avoiding labor bottlenecks will also reduce advanced nuclear costs, such as by eliminating downtime and workforce turnover between successive orders and projects. Sustaining a sufficient volume of orders for new advanced nuclear projects will help avoid such issues by generating continued demand for services and personnel.
8.6 State Laws Related to New Nuclear Energy Construction
As of February 2022, twelve states restrict or ban the construction of new nuclear generating stations. Minnesota has imposed an outright ban on new nuclear energy, while New York has a ban on nuclear power plants that is specifically limited to Long Island. California, Connecticut and Illinois will only permit new nuclear generation on the condition that nationwide waste disposal or reprocessing pathways are developed. State legislatures in Hawai’i, Massachusetts, Rhode Island and Vermont restrict new nuclear construction unless various conditions are met (e.g., legislature approval and/or approval by registered state voters). Some states (e.g., Oregon and Maine) condition new nuclear on both a waste disposal repository and approval by public vote.
In New Jersey, approval of new nuclear projects depends upon securing a finding from its Commissioner of Environmental Protection that disposal of radioactive waste material produced by a project “will be safe, conforms to standards established by the Nuclear Regulatory Commission, and will effectively remove the danger to life and the environment from such waste material.”
States with Major Limitations on Nuclear Construction
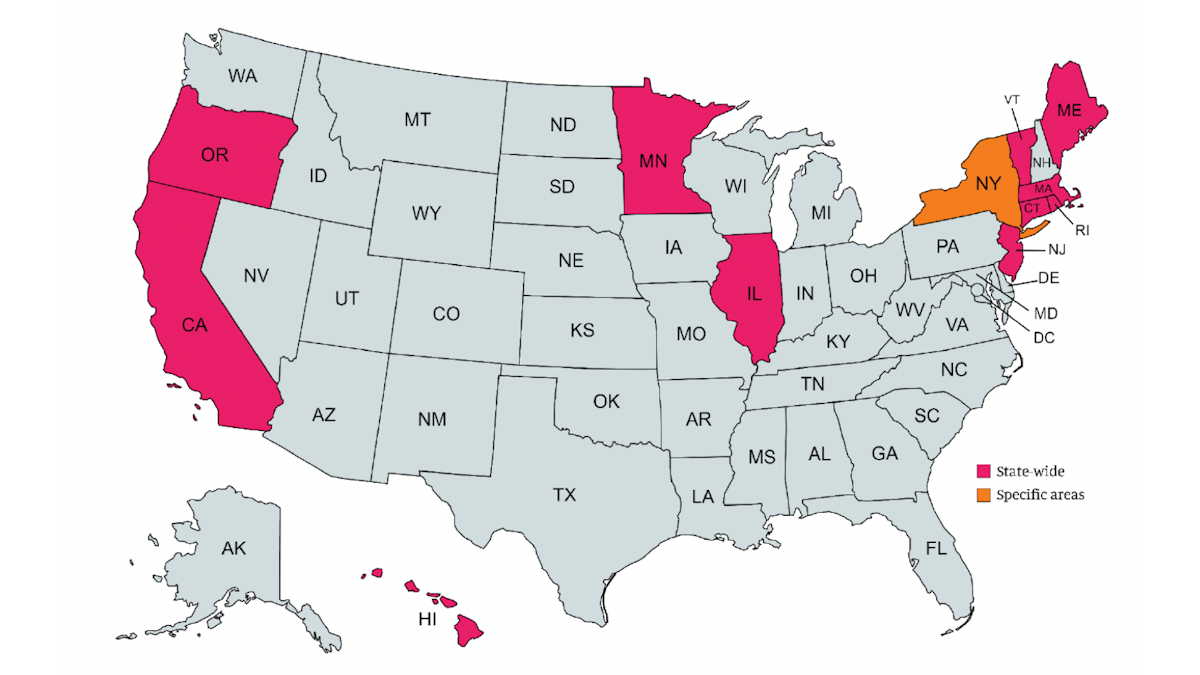
The conditions stipulated by these states are premised on the presumption that waste cannot be safely stored today. However, the historical record demonstrates that high-level waste has been routinely and uneventfully transferred to safe, secure, and stable interim spent fuel storage canisters for decades. Storage sites have been installed at operating and decommissioned nuclear plant sites across the country, including the decommissioned Oyster Creek Nuclear Generating Station in Forked River, New Jersey.
Self-imposed state obstacles to new nuclear deployment hamper state-level and national efforts to reduce carbon emissions and achieve climate goals. States in which nuclear power plants have been shut have seen rising carbon dioxide emissions, undermining efforts to reduce carbon emissions and meet clean air goals. As reported in February 2022: “a rise in emissions follows the closure of three nuclear facilities in Massachusetts, New York and Pennsylvania since 2019. While all three states have expanded their renewable energy generation, natural gas has largely filled the void left by shuttered nuclear facilities, prompting emissions to rise.”
Growing State Support for Nuclear Power
In contrast, many states are increasing support for nuclear power in consideration of the many advantages nuclear energy offers as an alternative to carbon-emitting fuels, a source of high-paying jobs, and a tax revenue generator. Some states recognized early that nuclear energy is a safe, clean source of energy. These states are better positioned to meet climate goals. For example, as early as May 2014, the Illinois legislature adopted House Resolution 1146, which “supports the state’s existing nuclear fleet and urges the federal government and the Midwest grid operator to adopt policies and rules to protect Illinois’s nuclear plants for the sake of the environment, the economy, and energy reliability.” Since that time, the state has enacted legislation (S.B. 2814 and S.B. 18) to establish a zero-emission credit program for five nuclear power plants: in Clinton, Quad Cities, Byron, Dresden, and Braidwood.
In April 2021, the Montana legislature passed a bill that removed an obstacle —voter approval — from the development of nuclear facilities, paving the way for nuclear projects in that state. House Bill 273repealed a 1978 law that required a majority of Montana voters to approve siting of any nuclear energy facilities in the State. The new law was enacted in May 2021, and that same month Montana’s Senate adopted a joint resolution to study the feasibility of advanced nuclear generation, including the economic viability of siting advanced reactors at closing coal plants.
On January 10, 2022, Indiana Senator Eric Koch introduced Senate Bill 271 to amend statutes governing electric utilities and adopt rules for siting small modular reactors in the state. That bill was signed into law on March 18, 2022. On January 19, 2022, Nebraska State Senator Bruce Bostleman introduced LB1100, proposing the appropriation of $1 million of federal funding received by the state from the American Rescue Plan Act of 2021 to study the feasibility of converting existing electric generation facilities into advanced nuclear power plants.
On February 7, 2022, West Virginia Governor Jim Justice signed a bill lifting a 1996 ban on nuclear project, emphasizing nuclear energy’s potential as a clean alternative to coal, which supplies 88 percent of electricity to the state. Also on February 7, 2022, Oklahoma State Senator Nathan Dahm authored SB 1794 proposing to direct the state’s Department of Environmental Quality to study the feasibility and establishment of nuclear facilities in cooperation with the Office of the Secretary of Energy and Environment on or before January 1, 2024.
Many policy makers, environmentalists, and energy researchers anticipate this trend to continue as energy demands grow and fossil stations are shut or repurposed to achieve clean air goals. For example, the Ohio House recently passed House Bill 434, “enact[ing] the Advanced Nuclear Technology Helping Energize Mankind (ANTHEM) Act by establishing the Ohio Nuclear Development Authority.” The bill is before the Ohio Senate for a vote. Putin’s invasion of Ukraine on February 24, 2022, is now propelling more urgent calls for nuclear energy in the United States and abroad to secure energy independence from Russia, a global supplier of oil and natural gas.
However, such efforts are not universal. In Colorado, partisan politics appear to be blocking efforts to explore nuclear energy as a strategy for achieving clean air goals. The Republican-backed Senate Bill 22-073 would have required the Office of Economic Development to study using small modular nuclear reactors as a carbon-free energy source that would contribute to the state’s goal of reducing overall carbon emissions. However, the Democratic-controlled State, Veterans, and Military Affairs Committee voted to postpone the bill indefinitely by a 3-2 party-line vote.
Economic Impacts of State Barriers to New Nuclear Deployment
State-imposed constraints to clean energy deployment in the United States may produce very real future economic consequences. The extent of those consequences is analyzed in this section.
Barriers to the deployment of clean, nuclear energy impact state economies. The modeled scenarios in this report build a substantial number of new advanced nuclear power facilities to meet both climate and least-cost constraints. As such, any legal limitations that prevent advanced nuclear construction could increase overall retail electricity prices.
Construction of nuclear energy facilities involves a direct investment of capital in the state, support for local businesses and industries, and the creation of both construction and permanent jobs. If restrictive state laws preventing new nuclear projects remain in place, an estimate of unrealized state-level capital investments as projected in the modeled scenarios can be assessed from the capital required for nuclear energy construction in those states.
Unrealized Capital Investment through 2040
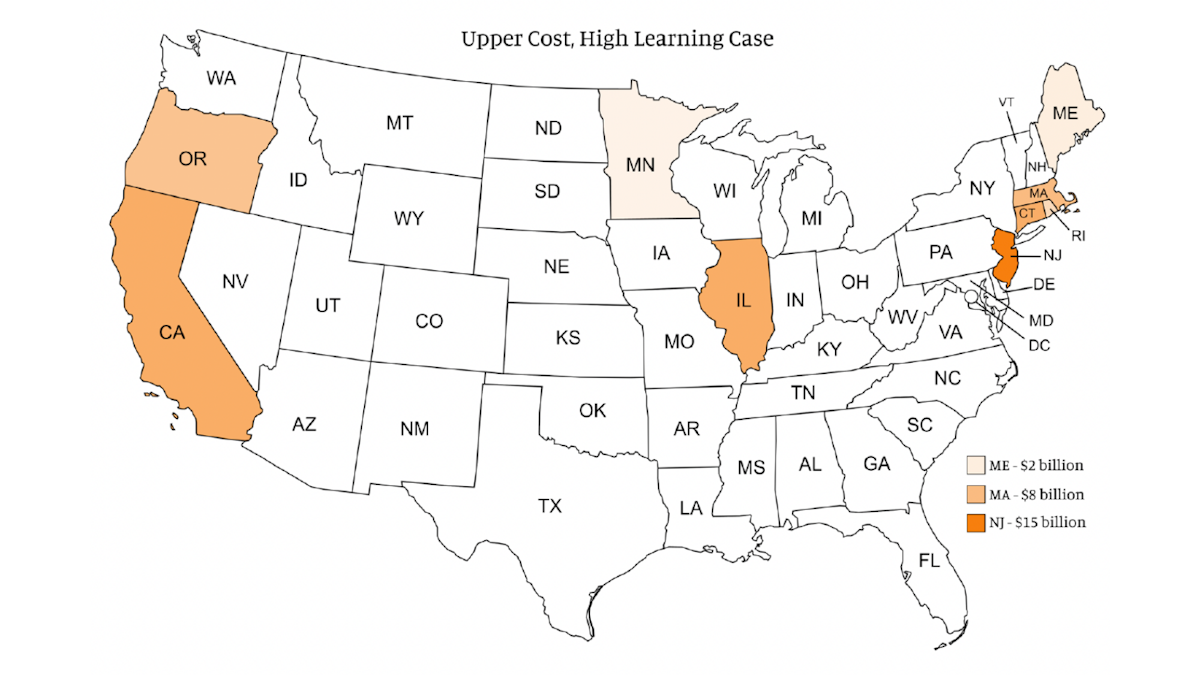
Based on the model results, states that have laws restricting new nuclear power plant projects may forgo local capital investments of $64 to $75 billion cumulatively between 2020 and 2040. In particular, New Jersey, California, and Illinois might relinquish substantial capital investment opportunities that might be unlocked if state-level restrictions were revised or repealed. These three states might otherwise benefit from advanced nuclear projects worth up to $15, $12, and $12 billion respectively. This analysis does not include unrealized capital investment in Hawai’i, which was not included in the model.
9. Mechanisms for Advanced Nuclear Public Policy Support
Policymakers possess numerous financial and non-financial opportunities to support the successful deployment of advanced nuclear power plants at scale. Policy mechanisms for financial support can help lower costs and reduce the financial risk associated with early projects while encouraging the growth of a robust industry that includes not only reactor developers but also upstream manufacturers and suppliers. Meanwhile, non-financial policy support can help facilitate power plant siting, train a skilled workforce, formalize management strategies for spent fuel, and improve the efficiency with which the United States advanced nuclear industry can secure customers internationally. Proactive public policy support across this broad range of issue areas will prove crucial for positioning the United States advantageously as a technology leader in advanced nuclear energy.
9.1 Direct Financial Support Mechanisms
Federal Loan Guarantees
Upfront capital investments will comprise much of the cost of advanced nuclear projects. Due to the higher financial risk associated with backing emerging advanced nuclear reactor deployments, financiers will likely expect higher interest rates for lent capital. Higher interest rates thus add to the cost of early deployment of advanced nuclear technologies.
To encourage capital investment into US advanced nuclear projects and to reduce project costs, federal programs like those administered by the US DOE’s Loan Programs Office (LPO) can guarantee repayment of loans for advanced nuclear projects, both reducing financial risks for investors and allowing project developers to secure capital at lower interest rates. Such federal loan guarantees can thus play a highly influential role in accelerating the domestic development of an advanced nuclear sector.
At the national level, the DOE LPO seeks to provide directed public support for energy innovation. The DOE LPO currently possesses the capacity to issue up to $40 billion in loans and loan guarantees to support a wide range of groundbreaking energy and energy infrastructure initiatives, with up to $10.9 billion in loan guarantees available for promising nuclear energy projects. This support has historically been extended to conventional nuclear projects such as the construction of Units 3 and 4 at the Vogtle Electric Generating Plant in Waynesboro, Georgia.
Demonstration and Cost Share
Publicly funded technology demonstration programs remain a primary driver to assist innovative and transformative research to reach commercial scale. Over the last 80 years, the Department of Energy and the world-leading system of US national laboratories have directly driven not only the development but also the demonstration of many new energy technologies nationwide. Demonstration programs represent a critical step in the innovation process by bridging the research and development process and full-scale commercialization of a technology. Public-private partnerships for demonstration projects reduce the burden on the government to solely demonstrate the technology. By participating in project cost-sharing, the government facilitates “buying down” financial risk, thereby reducing overall FOAK costs. Such demand-pull innovation policies have a demonstrated track record of success in commercializing innovative technologies in a variety of sectors.
The DOE’s recent opening of a new Office of Clean Energy Demonstrations (OCED) emphasizes the value of this public sector role in driving early deployment for emerging technologies. The OCED will seek to support a range of important technologies, such as carbon capture, clean hydrogen, grid infrastructure upgrades, and advanced nuclear demonstration. The recently passed Bipartisan Infrastructure Law specifically designated $2.5 billion in OCED funding to support two advanced nuclear reactor demonstration projects through the Advanced Reactor Demonstration Program (ARDP).
The ARDP is a more established but still recent program launched in 2020 to provide public support and help advanced nuclear developers secure and build their first projects. The ARDP currently supports 10 projects, with two full scale demonstration projects. One demonstration project will deploy four of X-Energy’s 80 MWe Xe-100 high-temperature, gas-cooled small reactors at the Columbia Generating Station in Washington state, currently home to an existing conventional nuclear power plant. The other demonstration project will involve building TerraPower’s Natrium 345 MWe sodium-cooled fast reactor, with an initial reactor slated for construction in Kemmerer, Wyoming at the site of the existing Naughton Coal Plant. Others ARDP projects include the Kairos KP-X/Hermes 50 MWe test reactor intended for construction at the Oak Ridge National Laboratory in Tennessee. Other deployment projects include the six-unit NuScale SMR project at INL and GE-Hitachi’s BWRX-300 design, at the Clinch River site in Roane County, Tennessee.
Tax Credits
Tax credits for renewable electricity generation are a well-established policy mechanism for encouraging the greater deployment of new domestic wind and solar capacity. Power produced by conventional and advanced nuclear reactors provides the same climate and air pollution benefits as other sources of clean energy. To promote wider adoption of clean electricity from a diverse array of sources, optimally-designed clean energy tax credits should be available on a technology-neutral basis. A future low-carbon electricity grid will rely upon an array of technologies, so nuclear power plants, geothermal facilities, and hydroelectric dams should similarly benefit from federal tax incentives intended to accelerate national clean power generation. Such federal tax incentives will further improve the economics of new advanced nuclear projects.
Tax credits require the entity to have a sufficient tax burden for credit to offset. Small organizations pursuing projects with no existing revenue, therefore no tax liability, often have to partner with another organization, which in turn takes some of the tax credit for the service. One option to avoid this issue is a direct payment of the tax credit to the entity. A proposed direct pay mechanism allows a taxpayer to treat tax credits that it has earned as an overpayment of taxes, allowing the tax credit to be received as a direct payment of cash in the form of a refund.
Subsidies
It is our view that technology-neutral subsidies are best employed to promote the accelerated early deployment of innovative clean energy technologies in a fair and efficient manner. As a promising set of clean energy sources that offer unique strategic and economic advantages for the United States, domestic advanced nuclear energy projects are strongly in the national interest and possess a good case for inclusion in any technology-neutral clean energy subsidy program.
In the long term, inefficient subsidies may discourage innovation and further improvements in efficiency, so such policies might be reconsidered in the future once these technologies have become more established.
Tax incentives for the Development of Advanced Nuclear and HALEU Supply Chains
Successful commercial deployment of advanced reactors will also depend upon the expansion of robust upstream supply chains, including factory manufacturing capabilities, production of specialized alloys, and components of the nuclear fuel cycle (uranium mining and milling capacity, fuel fabrication, and spent fuel and high-level waste processing and reprocessing facilities). Establishing sufficient capacity across these industries will support the commercial-scale buildout of advanced reactor designs, reducing technology costs due to faster rates of technological learning in the factory, improvements in the cost and availability of components, and more affordable HALEU fuel inputs.
Tax incentives can spur growth in these upstream and downstream sectors, promoting the development of additional advanced nuclear supply chain capacity beyond that the market alone would produce. Such policy measures would yield benefits not just during the early stage of advanced nuclear deployment, but well beyond as the industry enters successive stages of maturity and scale.
9.2 Supporting Policies and Programs
Support for Environmental Impact Studies and Pre-Qualification of Proposed Sites
Another means of helpful policy support for advanced nuclear would involve federal spending to identify promising sites for near-term deployment and conduct environmental impact studies (EIS) to assess potential candidate locations.
Such efforts would reduce costs for early deployment, first by obviating the need for the developer to fund the EIS, and second by reducing financial risks associated with potential rejection of an EIS. Additionally, pre-qualification of desirable sites for advanced nuclear reactors would accelerate the power plant planning timeline, further reducing costs and minimizing potential delays. Performing EISs in advance is thus an affordable policy measure that can meaningfully increase the efficiency of advanced nuclear deployment at no cost to environmental oversight.
Federal Procurement of and Pre-Orders for Advanced Nuclear Designs
Upstream supply chains for the domestic advanced nuclear industry will require a sufficient level of customer demand to become more established. The federal government is one of the largest single electricity purchasers. Federal procurement of electricity through contract or direct purchase of advanced nuclear projects will provide a strong market signal to industry, incentivizing the production of HALEU fuel, reactor components, and associated services. The public sector could drive early pre-orders of reactor projects by siting a number of small reactors or microreactors at military installations, government facilities and infrastructure, public universities, and other locations in service of state and national needs. Such public sector projects could help support the Biden administration’s Executive Order on Catalyzing Clean Energy Industries and Jobs Through Federal Sustainability, which directs the federal government to procure 100 percent of all electricity for federal operations using clean electricity, with at least 50 percent produced from 24/7 carbon-free generation.
Public policies could also support the development of specific nuclear manufacturing sectors and services. Development of a secure supply chain to ensure sufficient domestic HALEU supplies, for instance, would serve the national interest and intersect with any federal advanced reactor procurement efforts.
Workforce Education
Another key input for the advanced nuclear industry is an expert workforce. From fuel fabrication to power plant engineering, plant operations to spent fuel management, the future advanced nuclear sector will require specialized knowledge and skills that will be in increasingly high demand nationwide. Public support for higher education programs that train industry specialists can help ensure a sufficient supply of well-qualified labor, while public funding for academic research in nuclear engineering and nuclear science will expand the potential workforce with advanced degrees.
Investing in education and training can help prevent a shortage of skilled employees from constraining the growth of the advanced nuclear sector at large. At the same time, public funding in nuclear science and engineering will advance these fields of research, producing additional benefits for US technology leadership and bolstering other sectors of the American economy. Such support, if well designed, could also increase economic prospects for young Americans, particularly for students from poor backgrounds and minority populations.
Consent-Based Siting of Spent Fuel and High-Level Waste Storage and Reprocessing
The presence of robust back-end solutions for spent nuclear fuel will also encourage the growth of a robust and responsible US advanced nuclear sector. Public funding to support the solicitation and review of nationwide siting applications for spent nuclear fuel storage sites, high-level waste storage sites, spent fuel reprocessing plants, vitrification facilities, and similar components of the back-end nuclear fuel cycle can help address longstanding nationwide needs for such services and capabilities.
An effective consent-based siting process for spent fuel storage will help proactively address and mitigate potential public opposition to new advanced nuclear power projects. A competitive, consent-based site selection framework will also support local economic activity in communities that successfully bid to host facilities. By encouraging the development of new spent fuel storage and reprocessing sites, such federal programs may also help alleviate obstacles presented by several state laws that prohibit the construction of new nuclear power plants until more satisfactory permanent storage of spent fuel and high-level waste is in place.
Solutions for Export Control Challenges
Export sales of advanced reactors and SMRs abroad can enhance domestic commercial prospects for these technologies by accelerating technological learning, encouraging expanded factory production, and creating a stronger demand signal for industry actors. Active US competition in the international market for nuclear energy technology will help foster a stronger and more advanced domestic next-generation nuclear industry.
the United States imposes controls on the international transfer of civil nuclear power technology and reactors. These controls are governed by the DOE and National Nuclear Security Administration (NNSA), while the NRC and Department of Commerce provide assistance in multiple capacities. It should also be noted that nuclear export approvals are often required several years before any actual components are exported, as the approvals also govern the exchange of information and sensitive technical details.
The export of intangible nuclear technology and knowledge is licensed by the DOE through Part 810 agreements, which permit the sharing of digital blueprints, development of technical standards, and where applicable, sharing of proprietary designated information. Part 810 authorizations are provided by the DOE after concurrence from the Department of State, and consultation with the Department of Commerce, Department of Defense, and the NRC.
The NRC issues licenses for exports of “nuclear reactors and especially designed or prepared equipment and components for nuclear reactors” under 10 CFR Part 110.8(a). The Department of Commerce regulates the export of “dual-use” nuclear items, which are those with primarily commercial applications that also have proliferation risks. This regulation is performed under the United States Export Administration Regulations, through the Bureau of Industry and Security. The Department of State collects input from the Departments of Commerce, Defense, and Energy for assurances related to the Nuclear Cooperation Agreement (also called the “123 agreements”) with the international partner. These inputs are provided to the NRC to support Part 110 license approvals.
All three agencies tasked with export controls have reviewed their existing processes and determined that current practices are adequate to support advanced reactor technology exports with minor modifications, particularly to address safeguards. Nevertheless, the United States federal government can better support the export of US advanced reactors and SMRs abroad in several ways.
First, federal agencies should organize additional outreach efforts to the community of advanced reactor developers. Some advanced reactor developers are less experienced with the export control framework than more well-established nuclear vendors, and early communication and outreach to the emerging advanced reactor industry will help industry actors more capably navigate this process. Agencies should also proactively develop an integrated schedule of activities across all participating agencies to identify optimization opportunities that could reduce the lead time needed to secure export approval.
Second, the United States Department of State should proactively review its existing 123 agreements and develop new future nuclear cooperation agreements in close consultation with the relevant international partners to address potential obstacles to US nuclear exports. For instance, hesitancy over final responsibility for spent nuclear fuel could be addressed through arrangements in which the United States government and US vendors agree to re-import spent fuel for domestic long-term storage or reprocessing. Alternatively, the Department of State could work with foreign governments to develop arrangements for reprocessing of spent fuel in the partner country, or for the exporting of spent fuel to a friendly third-party country with reprocessing or storage capabilities. Taking the considerations of international partners into account, the United States government should also work with partner governments to explore more flexible agreement terms that permit uranium enrichment or fuel fabrication in partner countries.
Difficulties regarding project financing could be alleviated through a Build-Own-Operate or Build-Own-Operate-Transfer model in which US vendors construct and operate nuclear projects in the partner country and recoup investments through local energy sales, with the potential for the power plant to be transferred to the foreign government or a local investor at a later time.
Finally, federal agencies should work with peer governments and institutions abroad to support and accelerate the development of robust institutions, regulatory agencies, technical capabilities, and other key factors for successful nuclear cooperation among international governments.
Conclusion
Advanced nuclear projects can drive progress on United States climate goals and deliver hundreds of thousands of jobs nationwide. Deploying advanced reactors will create both local and national co-benefits, providing reliable, resilient electricity and generating local economic activity and public revenue for communities while strengthening energy security and promoting leadership in nuclear energy technology for America.
This report explores how a large-scale clean energy transition in the United States could drive construction of a significant quantity of new advanced reactors, with advanced nuclear providing 20-48% of domestic clean electricity by the year 2050. The magnitude of nationwide deployment even in high-cost scenarios highlights the high value that advanced reactors add to a decarbonized energy system by acting as a clean, firm source of power that complements variable renewable technologies like wind and solar to efficiently meet electricity demand. Able to co-generate clean, useful heat and steam to meet numerous community and industrial needs, advanced nuclear designs could ultimately support clean energy applications well beyond the electricity sector.
Successful establishment of a domestic advanced nuclear sector will only be possible with increasing capital investment starting from the present, with cumulative capital deployment reaching $150 to $220 billion by 2035. Cost drivers will play a critical role in determining the pace and extent of advanced reactor deployment, with early projects driving important cost improvements through learning-by-doing.
Overall, large-scale deployment of advanced nuclear reactors is now more of a question of capital and sound planning than one of technological preparedness. Proactive supporting policies like the modernization of nuclear regulatory frameworks, public-private partnerships, federal loan guarantees, and tax credits to support power plant and manufacturing supply chain projects will significantly accelerate near-term progress and expand long-term domestic technological potential. Policymakers and advanced nuclear developers will also need to overcome possible barriers, by ensuring adequate nuclear fuel supplies, promoting efficient project management, and developing a skilled sector workforce.
Advanced nuclear power presents a once-in-a-generation opportunity that has languished for decades. Yet the world is increasingly interested in the energy security, economic, and climate benefits of reliable and clean nuclear energy, deployment efforts are accelerating. If the United States does not also act soon to seize this opening, the probability is high that the American advanced nuclear industry will be left behind. On the other hand, if America can execute a proactive, planned advanced nuclear deployment strategy, then the United States can play a leading role in introducing a revolutionary new energy technology to the world at a critical moment in the global clean energy transition.
For access to the appendix and references, please download the PDF version of the report.
Download the Full Report >>>